1. Introduction
Volcanic activity on Earth and elsewhere is expressed through a variety of features including volcanic edifices (strato-volcanoes, shields, cones, domes, craters…), lava flows, intrusive features (dikes, laccoliths…), among others. The shape of surface features is known to result from complex, interrelated parameters such as the magma properties, eruption style, but also the planetary variables (e.g., gravity, presence of an atmosphere…) (e.g., [1]). On the Moon, large plains of basaltic composition referred to as lunar maria are most representative of surface eruptions, and volcanic edifices are rarer (e.g., [2]). Still, a range of volcanic constructs such as pyroclastic deposits, cones and domes are observed at various locations. The focus of this study are lunar domes which show various geometries, with diameters typically ranging from a few km to 30 km and slopes up to 9° [3,4]. Previous studies have used the dome geometries as an input for rheological models, in order to estimate the magma apparent viscosity [3-6]. In the present paper, we apply (and discuss) similar models to terrestrial analog domes from the Atacama Desert in northern Chile, for which we obtained ground truth through field sampling and analyses.
2. Geological context
The Andean volcanoes in the Atacama Desert offer an unique environment to test and improve existing mineral mapping techniques and rheology equations, as they are well preserved due to their relatively young (quaternary) ages, and to the Atacama desert hyperarid environment (e.g., [7]). Indeed, the Atacama Desert is the driest non-polar desert on Earth, and for this reason, it has already been previously studied as a Moon and Mars analog environment (e.g., [8]).
Five Atacama domes were studied from orbit, and three of them were sampled on the field. Dome dimensions as measured on ASTER DEM are presented in Table 1. Previous studies suggest these features were emplaced by monogenetic eruptions and comprise high K dacitic and rhyodacitic rocks [9, 10]. Collected samples are dominated by glass (~60 vol%), and phenocrysts of plagioclase (~20 vol%), hornblende (~7 vol%), biotite (~4 vol%), alkali feldspar (~3 vol%), orthopyroxenes (~1 vol%) and quartz. Dome bulk rock compositions were further determined by ICP-MS/ICP-OES analyzed at the SARM facility in Nancy, France. The bulk compositions of the domes are very similar to each other with SiO2 contents ranging from 66 to 68 wt%.
3. Dome rheology
Rheology, which refers to the flow properties of the lavas, is controlled by magma properties and especially viscosity. Viscosity is a function of multiple parameters such as the temperature, composition, gas content and degree of crystallization. Fluid dynamics laws have been utilized to develop first-order mathematical equations that tie magma viscosity to the geometry of a flow or edifice (e.g., [11]).
To the first order, dome eruptions can be considered as the extrusion of a Bingham fluid (the cooling magma) characterized by a yield strength τ and a plastic viscosity η. By applying similar rheologic equations to the ones in [2-6], the yield strength and apparent plastic viscosity of the Atacama domes has been estimated (Table 1).
In parallel, the non-Arrhenian Newton silicate melt viscosity is calculated using the dome bulk composition as an input in the models of [12] (Table 1). The difference between apparent and liquid viscosity was further used to calculate the packing fraction with the Einstein-Roscoe equation, as in [13] (Table 1).
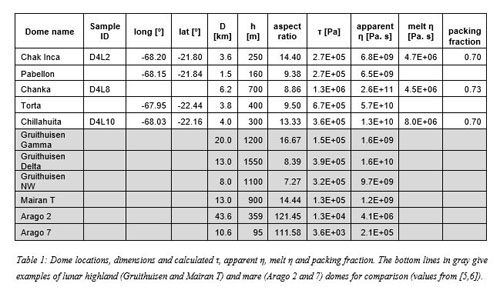
4. Discussion and Perspectives
We compare viscosity estimates obtained from remote sensing data (apparent viscosity) and sample analyses (liquid viscosity) for the domes and found that they differ by several orders of magnitude (Table 1).
A plausible explanation is that magma viscosity depends on several parameters (see above). The presence of crystals likely played a role in the elevated apparent viscosity values that we obtained, as suggested by the high packing fraction of ~0.7 (very close to the theoretical maximum packing fraction of 0.74), calculated for all domes.
Dacitic domes in the Atacama Desert have aspect ratios, yield strengths and apparent viscosities similar to the G class of lunar domes as reported by [4] (also referred to as highland domes). This class of domes include the Gruithuisen and Mairan edifices where elevated silica contents were previously reported (e.g., [5-14]. We thus argue that the Atacama domes may be a good analog for these lunar domes, which are likely made of felsic rocks, and could represent the source of the lunar granites/rhyolites and zircons found in the Apollo samples.
5. Acknowledgments
Support to JF from the CNES Luna APR, the LUE future leader and the CNRS Momentum program is much appreciated. This work has been also funded by the Comisión Nacional de Investigación Científica y Tecnológica (CONICYT-FONDAP 15090013/Centro de Excelencia en Geotermia de los Andes-Universidad de Chile). OGM and JF are grateful for support from the Claude Gay program of the French embassy in Chile.
6. References
[1] Whitford-Stark J.L. and J.W. Head (1982), Lunar and Planetary Science Conference, 8th, 2705-2724.
[2] Head III J.W., & L. Wilson (1992), Geochimica et Cosmochimica Acta, 56(6), 2,155-2,175.
[3] Wöhler C. et al. (2007), Icarus, 189(2), 279-307.
[4] Lena, R. et al. (2013), Springer Praxis Books.
[5] Wilson L. and J.W. Head (2003), JGR, 108, 5012.
[6] Schnuriger N. et al. (2020), PSS, 185, 104901.
[7] Ito G. et al., (2020), this meeting.
[8] Flahaut J. et al. (2017), Icarus, 282, 152-173.
[9] de Silva S.L. et al. (1994), JGR, 99, B9, 17,805-17,825.
[10] Taussi M. et al. (2019), JVGR, 373, 179-198.
[11] Hulme G. (1974), Geophys. Journal International, 39 (2), 361–383.
[12] Giordano D. et al. (2008), EPSL 271,123–134.
[13] Chevrel M.O. et al. (2013), EPSL, 394, 109-120.
[14] Glotch, T. D. et al. (2011), GRL, 38, L21204.