Transmission spectra have suggested that condensate clouds and/ or photochemical haze are present in the atmospheres of many temperate to warm exoplanets [1]. Aerosols can affect atmospheric composition directly through condensation and heterogeneous reactions, and indirectly by impacting the depth of penetration of photolyzing radiation, as well as the thermal structure and dynamics [2]. However, haziness may not be simply controlled by a single or a simple combination of planetary/stellar parameters [3,4]. We still need more observations, modeling work, and laboratory experiments to fully understand the complex physical and chemical processes that lead to hazy exoplanet atmospheres [5].
We have performed a series of laboratory atmosphere simulation experiments dedicated to the synthesis of haze analogues (tholins) under a broad range of atmospheric compositions and temperature (from 102× to 104× solar metallicity, 300–800 K) relevant to sub-Neptunes. In the solid organic material produced from a gas mixture designed to be analogous to a 103× solar metallicity exoplanet atmosphere at 400 K, thousands of molecular species with general chemical formulae C𝑐HhN𝑛O𝑜 were detected with very high-resolution mass spectrometry, possibly including some of prebiotic interest [6]. These measurements, performed on the soluble phase, represent only a fraction of the total sample. Here, we extend this previous study to focus on the difference between the soluble fraction, insoluble fraction and the bulk composition using a Fourier transform ion cyclotron resonance mass spectrometer coupled with a laser desorption ionization source (LDI-FTICR).
Laboratory analogues were synthesized at Johns Hopkins University in the PHAZER setup by exposing a gas mixture of 56% H2O, 14.7% He, 11% CH4, 10% CO2, 6.4% N2, and 1.9% H2 to an AC glow discharge. The gas mixture flows continuously through a stainless-steel reaction chamber. The produced particles are retrieved from the chamber’s inside wall, transferred to plastic vials and kept in an inert atmosphere, in the dark. More details about sample production and recovery can be found in [7].
A Fourier transform ion cyclotron resonance mass spectrometer (FTICR Solarix XR equipped with a 12 T superconducting magnet, Bruker available in the COBRA laboratory, at the University of Rouen, France) coupled with a laser desorption ionization source (LDI laser Nd:YAG×3 355 nm) was used to acquire ultra-high-resolution mass spectra (mass resolving power 𝑚∕Δ𝑚50% of 1,500,000 at m/z 150 and 500,000 at m/z 500). Analyses were performed in the m/z 100-1000 range in both positive and negative mode. To treat the data, we use the Attributor software that has been developed at IPAG for non-targeted analysis of mass spectrometry data, using IGOR Pro (WaveMetrics, USA). The detailed procedure used for the data treatment and validation is described in Cédric Wolters’ PhD thesis [8].
In order to determine the similarities between the soluble and insoluble fractions, we compare all the assignments and generate a Venn diagram, as shown in Figure 1. The Venn diagram shows that about one-third of the molecules assigned (7780 out of 23702) are present in all three phases. The majority of molecules detected in the bulk (14443) are found either in both the soluble and insoluble phase (7780), or only in the insoluble phase (5048). There is also a significant number of molecules unique to both the soluble fraction (3274), insoluble fraction (4993) or both (992), that are not found in the bulk, likely because of ionisation/sensitivity issues. This is in line with the findings of [9] for Titan tholins. Furthermore, the fractions present different overall characteristics in terms of unsaturation and content in heteroatoms. This may have some consequences for the ability of haze particles to act as cloud condensation nuclei in water-rich exoplanet atmospheres [9].
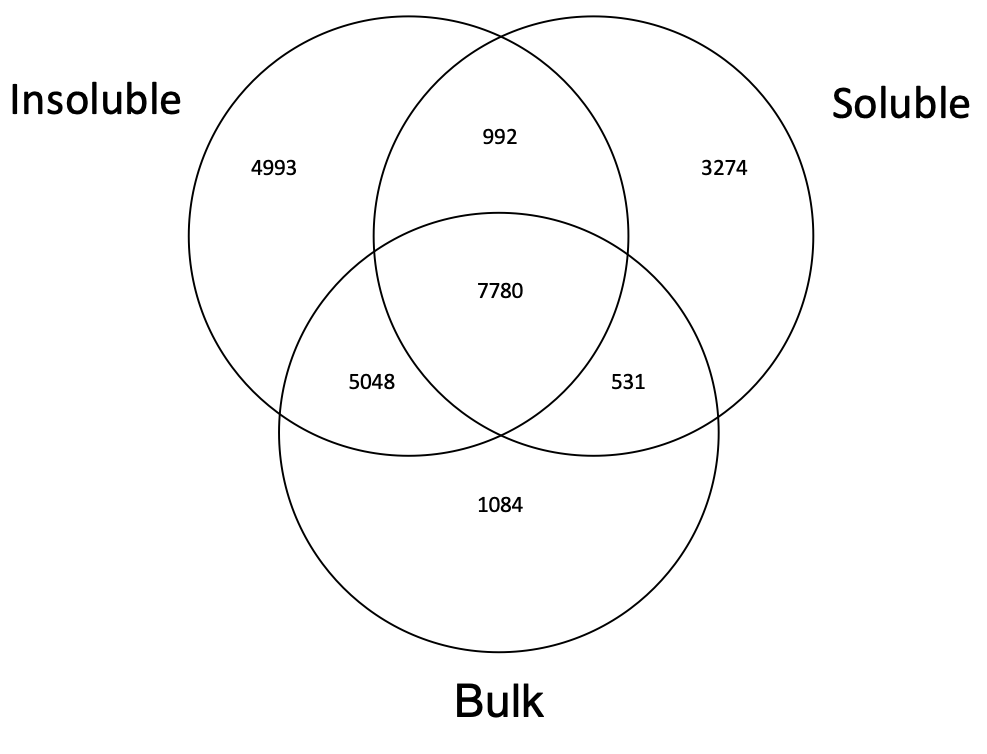
Figure 1: Venn diagram of the number of assignments for the soluble and insoluble fractions and bulk. Assignments common to each sample are shown in the intersecting spaces.
Acknowledgements
This work was supported by the French National Research Agency in the framework of the Investissements d’Avenir program (ANR-15-IDEX-02), through the funding of the "Origin of Life" project of the Univ. Grenoble-Alpes and the French Space Agency (CNES) under their Exobiology and Solar System programs. C. Wolters obtained a PhD fellowship from CNES/ANR (ANR-16-CE29-0015 2016-2021). C. He was supported by the Morton K. and Jane Blaustein Foundation. S.E. Moran was supported by NASA Earth and Space Science Fellowship Grant 80NSSC18K1109. Portions of this study were supported by NASA Exoplanets Research Program Grant NNX16AB45G. Access to the Centre National de la Recherche Scientifique (CNRS) FTICR research infrastructure Infranalytics (FR2054) is gratefully acknowledged.
References
[1] Madhusudhan, N., 2019. Exoplanetary Atmospheres: Key Insights, Challenges, and Prospects. Annu. Rev. Astron. Astrophys. 57, 617–663. doi:10.1146/annurev-astro-081817-051846.
[2] Mills, F.P., Moses, J.I., Gao, P., Tsai, S.M., 2021. The Diversity of Planetary Atmospheric Chemistry. Space Sci. Rev. 217, 43. doi:10.1007/ s11214- 021- 00810- 1.
[3] Dymont, A.H., Yu, X., Ohno, K., Zhang, et al., 2022. Cleaning Our Hazy Lens: Exploring Trends in Transmission Spectra of Warm Exoplanets. Astrophys. J. 937, 90. doi:10.3847/1538-4357/ac7f40.
[4] Estrela, R., Swain, M.R., Roudier, G.M., 2022. A Temperature Trend for Clouds and Hazes in Exoplanet Atmospheres. Astrophys. J. Lett. 941, L5. doi:10.3847/2041-8213/aca2aa.
[5] Gao, P., Wakeford, H.R., Moran, S.E., Parmentier, V., 2021. Aerosols in Exoplanet Atmospheres. J. Geophys. Res.-Planet 126, e06655. doi:10.1029/2020JE006655.
[6] Moran, S.E., Hörst, S.M., Vuitton, V., He, C., et al., 2020. Chemistry of Temperate Super-Earth and Mini-Neptune Atmospheric Hazes from Laboratory Experiments. Planet. Sci. J. 1, #17. doi:10.3847/PSJ/ab8eae.
[7] He C., Hörst, S.M, Lewis N.K., Yu, X., et al., 2018. Laboratory Simulations of Haze Formation in the Atmospheres of Super-Earths and Mini-Neptunes: Particle Color and Size Distribution, Astrophys. J. Lett., 856, L3. doi: 10.3847/2041-8213/aab42b
[8] Wolters, C., 2021. Caractérisation moléculaire d’échantillons organiques complexes par spectrométrie de masse et chromatographie en phase liquide. Ph.D. thesis. Université Grenoble Alpes.
[8] Maillard, J., Carrasco, N., Schmitz-Afonso, I., Gautier, T., Afonso, C., 2018. Comparison of soluble and insoluble organic matter in analogues of Titan’s aerosols. Earth Planet. Sc. Lett. 495, 185–191. doi:10.1016/j.epsl.2018.05.014.
[9] Yu, X., He, C., Zhang, X., Hörst, S.M., et al.., 2021. Haze evolution in temperature exoplanet atmospheres through surface energy measurement. Nature Astronomy 5, 822-831. doi:10.1038/s41550-021-01375-3.