Oral presentations and abstracts
Together with the recent Discovery and New Frontiers missions’ selections, a good number of ESA, JAXA, ISRO .. planetary missions and flight instruments are either in development or have been proposed and in review. Mission or instrument leads and/or team members are encouraged to present their missions or instruments for wider community awareness, lessons learned or for fostering future collaborations. Abstracts on concept planetary missions and instruments can also be considered for this session
Session assets
The JUpiter ICy moons Explorer (JUICE) is the European Space Agency (ESA) next large class mission to the Jovian system. The mission, scheduled to launch in 2022, will investigate Jupiter and characterize its icy moons, Callisto, Europa and Ganymede for a period of 3.5 years after a 7.5-year cruise to the planet. JUICE is planned to flyby Europa and Callisto, perform a high latitude tour of the Jovian system, and finally, at the end of the mission, it will orbit Ganymede at different altitudes inside the moon’s intrinsic magnetosphere.
While radiation is one of the major threats for all Space missions, in the Jovian system this problem is exacerbated due to the existent of very large fluxes of energetic electrons, with energies up to dozens of MeV, which can damage and eventually destroy the spacecraft systems. The existence of this electron population, and to a lesser extent of a proton and heavy ion population, is a consequence of Jupiter’s huge magnetosphere which can accelerate these particles to energies higher than those found in other known planetary magnetospheres. Although the Galileo mission, and to a lesser extent the Cassini, Pioneer and Voyager missions have provided ample information about the radiation environment in the Jovian, several questions about particle origin, acceleration mechanisms, Jovian-Solar magnetosphere coupling, and overall dynamics of the system still need to be answered with implications in magnetospheric physics, astrobiology and others, as well as in development of future manned and unmanned missions to both the inner and outer Solar System.
For these reasons, the JUICE mission will include the RADiation hard Electron Monitor (RADEM), a low power, low mass radiation monitor, that will increase the range of long-term spectral measurements acquired by the Energetic Particle Detector (EPD) aboard the Galileo spacecraft, from 11 to 40 MeV for electrons and from 55 to 250 MeV for protons. RADEM consists of three detector heads based on traditional silicon stack detector technologies: the Electron Detector Head (EDH), the Proton Detector Head (PDH), and the Heavy Ion Detector Head (HIDH), that will measure electrons from 0.3 MeV to 40 MeV, protons from 5 MeV to 250 MeV and Heavy Ions from Helium to Oxygen with energies from 8 to 670 MeV, respectively. Because the detectors have limited Field-Of-View, a fourth detector, the Directionality Detector Head (DDH) will measure electron angular distributions which can vary greatly along the Jovian System as observed by the Galileo spacecraft.
Although RADEM is a housekeeping instrument that will provide in-situ Total Ionizing Dose (TID) measurements and serve as a radiation level alarm, it has a broad scientific potential. Besides the Jovian system, the instrument will be fully operated during the cruise of the Solar System, which includes three Earth flybys, a Venus flyby and a Mars flyby, that offer additional scientific opportunities including but not limited to studying the cosmic ray gradient in the Solar System, characterizing Solar Energetic Particle (SEP) events, and others. In this work, we will present RADEM from a technical point-of-view, as well as the scientific opportunities that will be addressed by the radiation monitor during the JUICE mission.
How to cite: Pinto, M., Goncalves, P., Hajdas, W., and Socha, P.: The RADiation hard Electron Monitor (RADEM) for the JUICE mission, Europlanet Science Congress 2020, online, 21 Sep–9 Oct 2020, EPSC2020-311, https://doi.org/10.5194/epsc2020-311, 2020.
Multi-spacecraft missions after 2000 (Cluster II, THEMIS, Van Allen Probes, and MMS) have revolutionized our understanding of the causes, patterns and variability of a wide array of plasma phenomena in the terrestrial magnetospheric environment. ESCAPADE is a twin-spacecraft Mars mission concept that will similarly revolutionize our understanding of how solar wind momentum and energy flows throughout Mars’ magnetosphere to drive ion and sputtering escape, two processes which have helped shape Mars’ climate evolution over solar system history.
ESCAPADE will measure magnetic field strength and topology, ion plasma distributions (separated into light and heavy masses), as well as suprathermal electron flows and thermal electron and ion densities, from coordinated elliptical, 200 km x ~7000 km orbits. ESCAPADE are small spacecraft (<150 kg), traveling to Mars via solar electric propulsion as a rideshare with the Psyche metal-asteroid mission in August 2022. ESCAPADE’s strategically-designed 1-year, 2-part scientific campaign of temporally and spatially-separated multipoint measurements within and between the different regions of Mars’ diverse plasma environment, will allow the cause-and-effect of solar wind control of ion and sputtering escape to be unraveled for the first time. Figure 1 shows ESCAPADE’s orbits within a hybrid simulation of the solar wind interaction with Mars, where the color scale represents ion velocity, blue lines are magnetic field, while white lines are sample proton trajectories and spacecraft orbits.
ESCAPADE is due to complete its preliminary design review in August 2020, thereafter moving toward build, test, integration and launch two years later. We will report on science goals and objectives, mission design challenges, and provide a status update.
How to cite: Lillis, R., Curry, S., Luhmann, J., Ma, Y., Barjatya, A., Whittlesey, P., Livi, R., Larson, D., Xu, S., Russell, C., Fowler, C., Brain, D., Thiemann, E., Withers, P., Modolo, R., Harada, Y., and Berthomier, M.: ESCAPADE: Coordinated multipoint measurements of Mars' unique hybrid magnetosphere, Europlanet Science Congress 2020, online, 21 Sep–9 Oct 2020, EPSC2020-511, https://doi.org/10.5194/epsc2020-511, 2020.
Abstract: The study of exoplanets is essential to expanding our understanding of solar system formations, as well as, the development of life in the universe [1]. Unfortunately, with the vast distances between stars, we observe limited aspects of these exoplanetary systems by telescope. With the recent discovery of two interstellar objects (ISOs), one volatile-poor (1I/’Oumuamua [2]), and one volatile-rich (2I/Borisov [3]), we can detect and observe exoplanetary material bound for our solar system. We propose a new mission concept (Bridge) to flyby a yet-to-be-discovered ISO as it passes through our inner solar system. Bridge is feasible under NASA’s New Frontier’s class-mission; however, it will require changes to future NASA Announcements of Opportunity (AO) to provide long-term spacecraft storage and rapid launch response capabilities. While other ISO mission concepts require long-term missions as the ISO encounter occurs outside our solar system, Bridge is designed for an ISO encounter within 0.7-2 AU; streamlining the architectural design. Bridge would provide a unique opportunity to gain insight into physical, chemical, and biological differences between our solar systems as well as transport of planetary materials between them. An active community of ground-based surveys is key to catching potential targets. NASA ground-based telescope campaigns are awarded independently of mission AO. Providing an option to include ground-based observation time to secure the needed resources for optimum mission success, would be advantageous for these once-in-a-lifetime encounters. Bridge was designed during NASA’s 31st Planetary Science Summer Seminar [4].
Science goals & objectives: Bridge has two science goals: (1) determine whether prebiotic chemical ingredients can be transported between stellar systems and the interstellar medium, and (2) determine if interstellar objects form via the same processes as objects within our solar system. To address the first goal, Bridge would look for spectral signatures from CH, N2, polycyclic aromatic hydrocarbons, and tholins. To address the second goal, Bridge would compare its elemental abundances, isotope ratios, and relative abundances of noble gases to those of other solar system objects and presolar grains (e.g. [6]). Bridge would also look for the presence of ices, the molar abundances of minerals, and the morphological properties of the ISO to 10 m resolution in order to determine if the ISO is similar to any objects in our own solar system.
Science payload: Bridge’s instrument payload can address the aforementioned science goals and objectives regardless of the specific properties of the target ISO. Bridge utilizes a remote sensing suite consisting of a mid-infrared spectrometer, a near-infrared spectrometer, an ultraviolet/visible spectrometer, and a visible light camera. Bridge also employs a battery-powered guided impactor, based on the impactor from Deep Impact [7], to expose the ISO’s interior material. Table 1 lists the instruments and their properties. The high relative encounter velocities of our mission preclude direct in-situ sampling. All instruments in our selected payload are based on previously flown instruments.
Mission design: Bridge is designed to flyby a yet-to-be-discovered ISO. Multiple telescopic survey facilities exist that scout the sky for Near-Earth Objects, comets, and possible ISOs. It is estimated that ≈0.2 ISO detections should occur per year, and when future survey telescopes such as the Large Synoptic Survey Telescope become operational, the number of ISO detections should improve to ≈1 per year [8]. Bridge is designed to wait in storage on Earth until a suitable ISO is detected.
Bridge requires three criteria to be satisfied for a successful ISO flyby: (1) the ISO must pass through the ecliptic plane at a distance between 0.7 and 2.0 AU from the Sun (green region in Figure 2), (2) the relative encounter velocity must be less than 70 km/s, and (3) the total launch C3 is less than 60 km2/s2 for the intercept maneuver, which is achievable given the mass of Bridge and an Atlas V 431 launch vehicle. With these criteria, Bridge could encounter 65% of ISOs whose orbital characteristics are predicted by [1]. Figure 2 illustrates a hypothetical encounter with 1I/’Oumuamua that meets all the aforementioned criteria.
In a 70 km/s flyby scenario, the impactor would be released approximately eight hours before the main spacecraft achieved its closest approach. Fifteen minutes later the main spacecraft would perform a deflection maneuver, steering it to a safe closest approach distance of 8000 km. Thirty seconds prior to impact, the IR spectrometer and visible camera would observe the surface composition. The visible camera would capture a video at the time of the impact, at which point the UV spectrometer would image the ejecta plume flash. Following the impact, the spectrometers would continue to take measurements of the plume. All critical science data would be transmitted before the spacecraft’s closest approach to the ISO ninety seconds after impact. The complete encounter timeline is shown in Figure 3.
Recommendations: To enable Bridge in response to the unique and ephemeral nature of ISOs, several technological advancements and policy changes must occur. This includes: improved ground detection capabilities of small bodies, infrastructure to store a spacecraft in a launch-ready state, rapid launch response, and the opportunity to propose a mission that requires these capabilities. The planetary science community must advocate for language explicitly permitting mission architectures that include storage and rapid launch in future NASA New Frontiers, Discovery, and SIMPLEx AO.
Acknowledgments: This work was supported by NASA’s Planetary Science Division and NASA’s Radioisotope Power System program. The information presented about the Bridge mission concept is predecisional and is provided for planning and discussion purposes only. We thank JPL’s A-Team and TeamX for concept development support, and Leslie Lowes and Joyce Armijo for programmatic support.
References: [1] D. Seligman and G. Laughlin. AJ.,155(5):217, 2018. [2] K.J Meech et al. Nat., 552(7685), 2017. [3] P. Guzik et al. Nat. Astronomy, 2019. [4] C.J. Budney et al. LPSC 50, (3225), 2019. [5] National Research Council. The Ntl. Acad. Press, 2011. [6] A. Davis. Proc. of the Ntl. Acad. of Sci.,108(48):19142–19146, 2011. [7] M.F. A’Hearn et al. Sci.,310(5746):258–264, 2005. [8] D.E. Trilling et al. ApJ., 850(2):L38, 2017.
How to cite: LLera, K. and the NASA 31st PSSS group: Bridge To The Stars: A Mission Concept And Policy To Enable An Inner Solar System Encounter With An Interstellar Object, Europlanet Science Congress 2020, online, 21 Sep–9 Oct 2020, EPSC2020-575, https://doi.org/10.5194/epsc2020-575, 2020.
1. Introduction.
The Moon is no longer a goal in itself but a necessary step for the conquest of space. In this work we focus in the composition of Lunar meteorites as the only objects alongside Apollo, and Luna collected samples, that the scientific community has available to first-hand analyze the Moon. Future Artemis sample return missions will provide new samples to continue learning from our satellite. Given its proximity to our planet, our satellite is an ideal planetary body to use it as a space camp for new technology, to establish a space base, and to test space mining. The continuous depletion of Earth resources put special importance on the exploration of extraterrestrial natural resources potential and the feasibility of its exploitation. After many robotic and manned missions, the utilization of lunar resources has been studied for a long time. In-Situ Resources Utilization (ISRU) refers to the generation of materials (for construction, life support, or as propellants) from the available resources on a celestial body that otherwise, would have needed to be brought from Earth [1].
2. Analitical Techniques and Samples
For the present work, four different Lunar achondrites (Lunaites) were studied: Dhofar 1084, Jiddat al Harasis 838, Northwest Africa 2700 and 11444, and Miller Range 090031 (abbreviated in Table 1). Thin sections of each meteorite allow us a characterization of the mineralogy using SEM/EDX and optical microscopy (Fig. 1). In addition, XRD measurements on capillary powder samples of the meteorites are made using a powder diffractomer equipped with a Mo X-ray souce (λ=0.709 Å). This experimental configuration allows us to minimize preferential orientation effects as well as to significantly reduce the X-ray fluorescence signal from Fe, relative to XRD measurements performed with a standard Cu X-ray tube. Finally we perform ICP-MS and ICP-AES of the samples using a similar procedure that in our previous studies of meteorites [2]. Meteorite specimens studied so far are listed in Table 1.
Table 1. Lunar meteorites under study, type, probable origin and Total Known Mass (TKM).
3. Discussion
Like the Lunar rocks, Lunaites provide valuable information about the chemical and mineralogical complexity that can be found in the surface of the Moon. Despite that we don’t know exactly their origin in the Lunar surface, these relatively small rocks provide clues on the processes going on in the surface of the Moon. Among the most fascinating meteorites, JaH 838 is a mingled regolith breccia presenting mare and KREEPy clasts, together with the products of thermal processing: High-Al Si-Poor (HASP) glasses with chondritic metal grains. Another one is the complex melt breccia NWA 11444 containing a wide variety of angular fragments (gabbros and basalts) and variable amounts of flow-banded glass. Crystal fragments consist predominantly of plagioclase, pyroxene, and olivine. Finally the rock also contains a few percent of Fe,Ni metal grains probably reminiscence of the projectiles sculpting the rock (see Fig. 1).
Fig. 1. A kamacite grain set into an anorthite-rich matrix of NWA 11444.
4. Conclusions.
From our study and having into account our current knowledge of the chemical and mineralogical lunar resources which can be realistically used for ISRU, the following resources are considered [3,4]:
- Metals hosted in the lunar regolith are mostly due to the continuous impact of chondritic and metallic projectiles with the Lunar surface. As a consequence, the regolith is enriched in minerals such as pyroxene, olivine, ilmenite and native metals such as Fe and Ni. In addition, it can be found hydrated minerals and rare-Earth elements. As most metals are found in the form of oxides (as well as some pure kamacite), it makes their extraction costly energy-wise as these components tend to be chemically stable [3], but their potential use for producing spacecraft parts and in-situ repairs make them more attractive. On the other hand, the extraction of oxygen is especially interesting from a biological point of view: future missions may utilize it for the production of water and other life support processes.
- Water, probably to be found as ice inside permanently shadowed craters of the polar regions, could be used as rocket fuel and to support life in a Moon base. On the other hand, some regions of the Moon have been hit by carbonaceous chondritic asteroids, rich in clay minerals. These hydrous minerals contain absorbed and bound water. If heated at temperatures ∼100-150 ºC absorbed water can be released and bound water at ∼300 ºC [4].
- Carbon and other organic compounds are also common in regolith-rich regions of the Moon. There is a dominant flux of CM chondrites [5] that after impact dehydrate and end up as “graphitized” clasts observed in regolith breccias [6]. Furthermore, it has been theorized of an uncertain amount of hydrocarbons could be used for the production of much complex polymers, resins and plastics [1].
- Finally, solar wind volatiles become also implanted in the Lunar regolith: H, N, C and in particular the He-3 isotope, rare to find on Earth and key for future developments in nuclear fusion.
Conclusions
Lunar meteorites are valuable samples teaching us about the processes going on over the Moon, at the same time that provide clues on the most important minerals for mining. Many different initiatives on how to tackle Lunar resources are taking place: from 3-D printers that previously construct the necessary infrastructure for lunar mining, the utilization of autonomous robots and obviously, the mingle of different proposals according to the exploitation stage. The Lunar surface provides a lot of valuable materials, but a precise know-how is required to successfully exploit them. Then, a careful study of Lunar samples and meteorites will provide significant progress in optimizing ISRU.
Acknowledgements
The authors acknowledge financial support from the Spanish Ministry (PGC2018-097374-B-I00).
References
How to cite: Trigo-Rodríguez, J. M., Mas-Sanz, E., Ibáñez-Insa, J., and Alonso-Azcárate, J.: Moon in-situ resources: clues from the study of Lunar achondrites in preparation for Artemis sample return missions, Europlanet Science Congress 2020, online, 21 Sep–9 Oct 2020, EPSC2020-714, https://doi.org/10.5194/epsc2020-714, 2020.
Introduction: The search for evidence of life or its processes on Mars takes on two major themes: 1), the identification of environments that have or once had the potential to harbor life (habitability); and 2), the detection of morphological or chemical features suggestive of extinct or extant life (biosignatures). Compositional heterogeneity at the mm-to-100µm scale can reveal geological processes indicative of past or present habitability, and morphological and compositional heterogeneity on a similar length scale can provide evidence of life’s processes. The Mapping X-ray Fluorescence Spectrometer (MapX) is an arm-based in-situ instrument designed to identify these features on planetary surfaces [1].
Instrument Description: MapX is a full-field elemental imager capable of analyzing samples in situ without sample preparation. MapX has no moving parts, a 1-cm depth of field, and is designed to utilize 244Cm radioisotope sources, eliminating the need for a High Voltage Power Supply or X-ray tube. Figure 1 shows a schematic of the instrument, which consists of X-ray / ϒ-ray / α-particle sources, a focusing optic, and a CCD. The focusing lens is an X-ray micro-pore optic (MPO) which focuses X-ray photons 1:1 onto the CCD. The MPO has a large depth of field (~1cm) allowing rough unprepared surfaces to be imaged with minimal loss of resolution. The CCD is read out fast enough (several frames per second) so that each pixel records either a single photon from the sample or background. The number of electron hole pairs generated in a single pixel is directly proportional to the energy of the X-ray photon, and after summing a large number of individual frames, an XRF spectrum is generated for each pixel of the CCD. Each individual 0.3 sec. frame is a complete image; however many frames are necessary to produce quantifiable XRF spectra. Longer collection times will allow for improved signal to noise, but in the event a collection is interrupted the partial data will still yield a complete image. Downlinked data products include: Elemental maps 11<Z<40, instrument-selected [2] Regions of Interest (ROI) having common compositions, and quantifiable XRF spectra from ROI.
Figure 1. Schematic representation of MapX.
Example MapX datasets: rough surfaces, polished stubs, or petrographic thin-sections were imaged first on an EDAX commercial laboratory instrument (~50 µm resolution), then on MapX-III, a third generation prototype of MapX (~150 µm resolution). MapX-III data are collected in vacuum; on Mars, images will be collected at ambient pressure.
Figure 2 shows a partial MapX dataset collected from a quartz sandstone decorated with hematite crystals (similar to magnetite crystals observed on the surface of mudstones by the MSL Curiosity rover). The instrument nominally collects ~10,000 0.3 second images into an HDF5 data cube. An unsupervised machine learning algorithm resident on the instrument computer produces ROI comprised of common elemental compositions. Quantifiable XRF spectra are generated from each ROI, which are analyzed off-line using a fundamental parameters technique. ROI compositions are used in conjunction with the RRUFF database [3] to determine putative mineralogy.
Figure 2. Hematite crystals on quartz sandstone. a). image of sandstone fragment, scale bar = 1 cm; b). RGB elemental image from EDAX commercial instrument, Fe=Red, Si=Green, K=Blue; c). RGB elemental image from MapX-III prototype, same color scheme as b); d). instrument selected ROI, H = hematite, QS = quartz sandstone; e). XRF spectra from yellow ROI (hematite) and purple ROI (quartz sandstone).
Figure 3 shows a partial MapX-III dataset collected from a brecciated basalt infilled with hydrothermal carbonate cements from Spitsbergen, Norway (analogous to Comanche Carbonate reported from MER).
Figure 3. Basalt breccia cemented by carbonate. a). image of basalt breccia, scale bar = 1 cm (note: the image shown is the obverse of the side used for elemental imaging); b). RGB elemental image from EDAX commercial instrument, Fe=Red, Ca=Green, Mg=Blue; c). RGB elemental image from MapX-III prototype, same color scheme as b); d). instrument selected ROI, B = basalt breccia, C = Carbonate, MC = magnesian carbonate; e). XRF spectra from ROI.
Figure 4 shows a partial MapX-III dataset collected from a polished stub of 1.9 Gyr old Gunflint Chert Stromatolite (Schrieber Beach locality). While the compositional features in the rock are <50µm, the elemental images, ROI and XRF spectra returned by MapX-III are sufficient to identify this rock as a chert with preserved carbonates displaying stromatolitic features, deserving of further in-situ analysis or return to Earth.
Figure 4. Precambrian Gunflint Stromatolitic Chert. a). image of Gunflint Chert, scale bar = 1 cm; b). RGB elemental image from EDAX commercial instrument, Fe=Red, Ca=Green, Si=Blue; c). RGB elemental image from MapX-III prototype, same color scheme as b); d). instrument selected ROI, C = Chert, FC = Ferroan Carbonate; e). XRF spectra from ROI.
MapX Flight Instrument. Figure 5 shows cut-away and solid 3D views of the MapX camera head, containing the sources, imaging optics, CCD and camera electronics (“Arm Unit”). A second processing unit (“RAMP Unit”) is located in the body of the rover and contains the computer processor, communications and instrument control software. Table 1 shows the proposed mass, volume and power requirements of the instrument as well as survival and operating temperatures.
Figure 5. 3D models of the MapX flight instrument. MapX. Right: Rendering of the arm mounted instrument in a flight like configuration.
Acknowledgements: This work was supported by the Ames Center Investment Fund (CIF), NASA’s PICASSO (13-PICASSO-0111) and MatISSE (16-MATISE16_2-0005) programs, and the NASA Postdoctoral Program.
References: [1]. Walroth, R.C. et al. (2019). 9th Intl. Conf. on Mars, abstr. #6329. [2]. Walroth, R.C. et al. (2019). AbSciCon abstr. #142-177. [3] Lafuente B, Downs R T, Yang H, Stone N (2015). The power of databases: the RRUFF project. In: Highlights in Mineralogical Crystallography, T Armbruster and R M Danisi, eds. Berlin, Germany, W. De Gruyter, pp 1-30 (link)
How to cite: Blake, D., Walroth, R., Sarrazin, P., Bristow, T., Gailhanou, M., Downs, R., and Thompson, K.: MapX: An In-situ X-ray µ-Mapper for Habitability and Biosignature Studies, Europlanet Science Congress 2020, online, 21 Sep–9 Oct 2020, EPSC2020-70, https://doi.org/10.5194/epsc2020-70, 2020.
Radar for Icy Moons Exploration (RIME) is expected to sound the crust of the Jovian moon Ganymede down to a depth of 9 km. The presence of dust in the ice and a fragmented crust could give rise to both attenuation and volume scattering, potentially affecting radar propagation. We have performed simulations in three dielectric scenarios for dusty inclusions to estimate the two-way attenuation. The contribution of volume scattering has been evaluated in terms of two-way losses as a function of scatterer radius and porosity. Results show that both phenomena will not affect significantly the performance of RIME in the radar sounding of Ganymede.
Introduction
Thanks to the low attenuation of radio waves in the ice, the next ESA JUpiter ICy moons Explorer (JUICE) mission will host onboard the 9 MHz Radar for Icy Moons Exploration (RIME) (Bruzzone et al., 2013), and its main focus will be an extensive exploration of Ganymede. Radar performances on Ganymede have been recently evaluated considering the effect of surface clutter (Berquin et al., 2013; Ilyushin, 2014) or subsurface medium attenuation and volume scattering (Di Paolo et al., 2014; Heggy et al., 2017; Di Paolo et al., 2018). The latter quantities govern the penetration of the radar signal, and depend both on dielectric properties of the subsurface medium and geological structures (e.g., cracks, pores). Because of the dust present inside the icy crust of Ganymede, and its high attenuation, the dielectric properties of silicate dust result one of the most important ingredients for the estimation of radar performances. Here we present the evaluation of the two-way attenuation of a 9 MHz radar signal considering different dielectric models for dust inclusions. Furthermore, the evaluation of the losses associated to volume scattering in the first kilometers of fragmented ice are evaluated.
Methodology
We consider a 10 km thick crust, for which thermal and dust content profiles have been taken from Heggy et al. (2017), taking into account the dust percentages for bright and dark terrains. A porosity varying from 0 to 20% is considered. A value of 2 km is fixed for the brittle-ductile transition; above such depth, the presence of fragmented ice could give rise to volume scattering in the radar propagation. For the dielectric properties of ice, we consider the measurements of Kawada (1978) as a function of temperature. As a contaminant we consider different silicates, whose dielectric properties are reported in Table. The permittivity values for models 2 and 3 have been extracted from the original values for porous media by using an inverse Bruggeman formula.
Model # | Reference | Material | Permittivity |
1 | Nunes & Phillips (2006) | Shergottite | 8.8 + 0.017i |
2 | Heggy et al. (2012) | LL5 chondrite | 7.2 +. 0.033i |
3 | Heggy et al. (2012) | H5 chondrite | 9.0 + 0.204i |
The permittivity of the three-phase mixture (ice+dust+air) has been evaluated following a mixing rule for spherical inclusions (Sihvola, 1999):
Finally, from the temperature profile and temperature-dependent dielectric permittivity, two-way attenuation has been evaluated following Di Paolo et al. (2016).
The presence of a layer of fragmented ice could give rise to volume scattering inside the voids. We evaluate the scattering efficiency in the Mie regime as a function of pore radius, following the procedure of Deirmendjian involving hyperbolic functions (Ulaby et al., 1986). Finally, following Di Paolo et al. (2018), we calculate the volumetric extinction along the optical depth and the two-way losses as a function of porosity and pore radius.
Results
The two-way attenuations are reported in Fig. 1. If a high-attenuation silicate is present (model 3), deep radar penetration could be prevented. Nevertheless, the radar sounding of the first kilometers, where the main geological horizons are located, will be not affected even in the high-attenuation scenario. Figures 2-4 report the two-way losses due to volume scattering as a function of pore radius and porosity, for both bright and darks terrains, in the dielectric scenarios considered. The color scale has been saturated at 55 dB, the estimated dynamic range of the radar (Heggy et al., 2017). Depending on the dielectric model, the threshold value for significative volume scattering losses is reached for a 2.5-3.5 m pore radius.
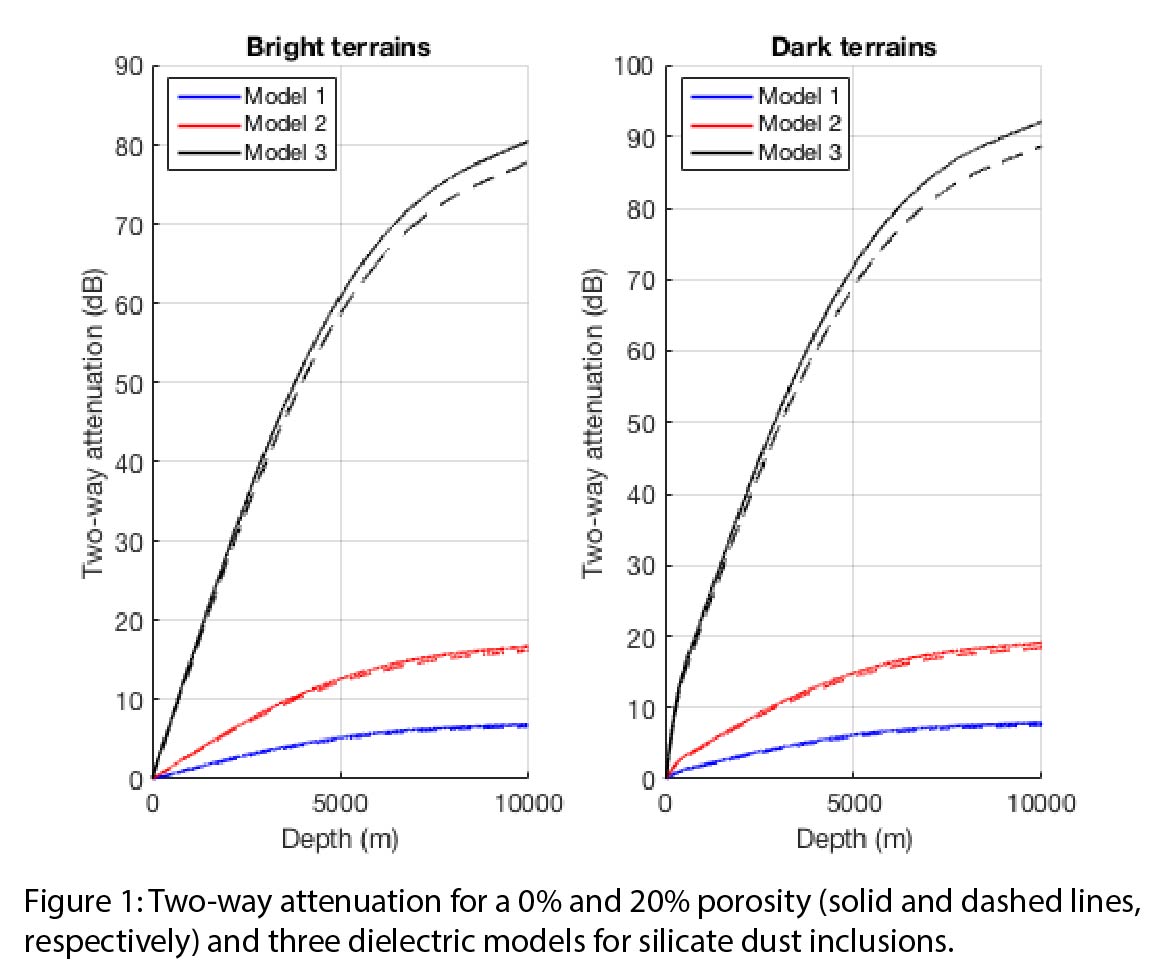
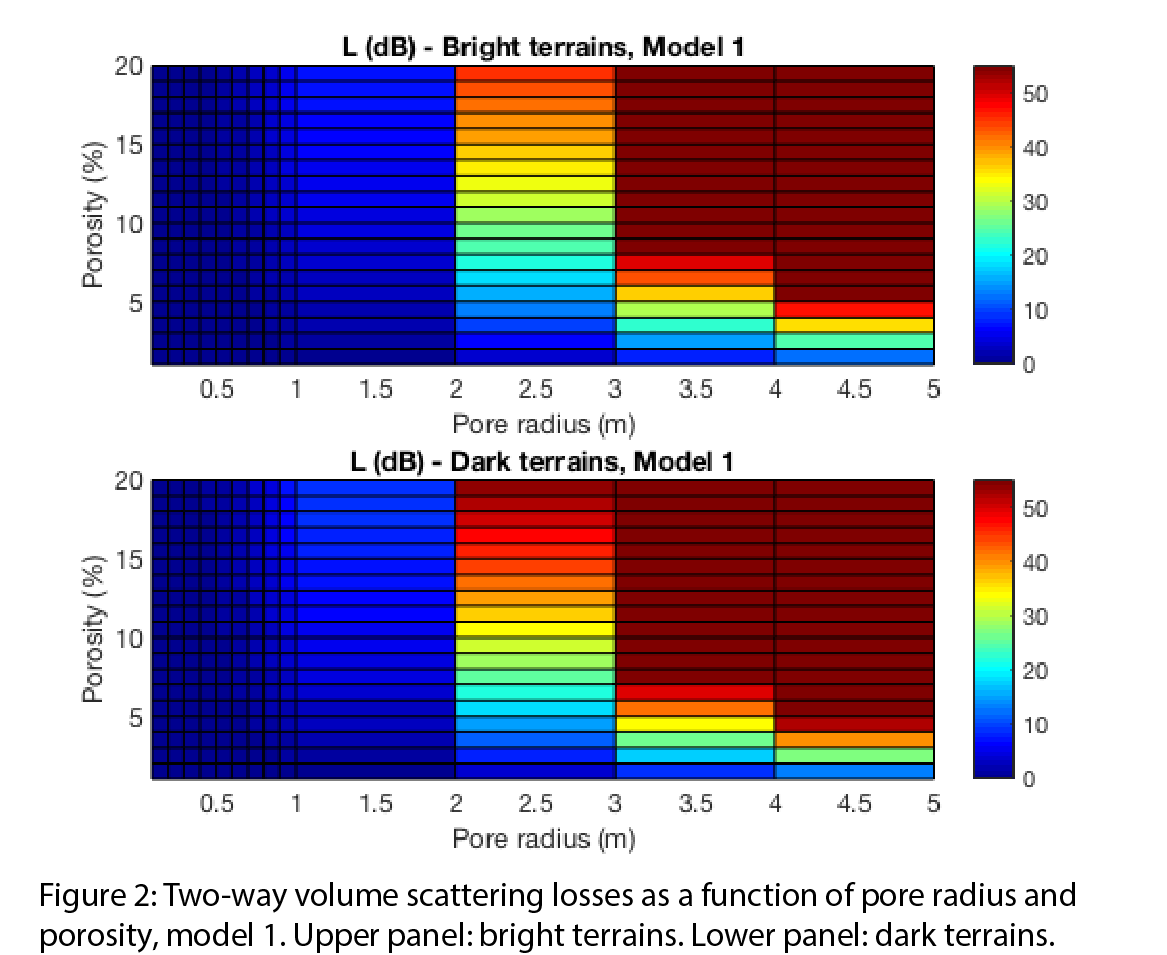
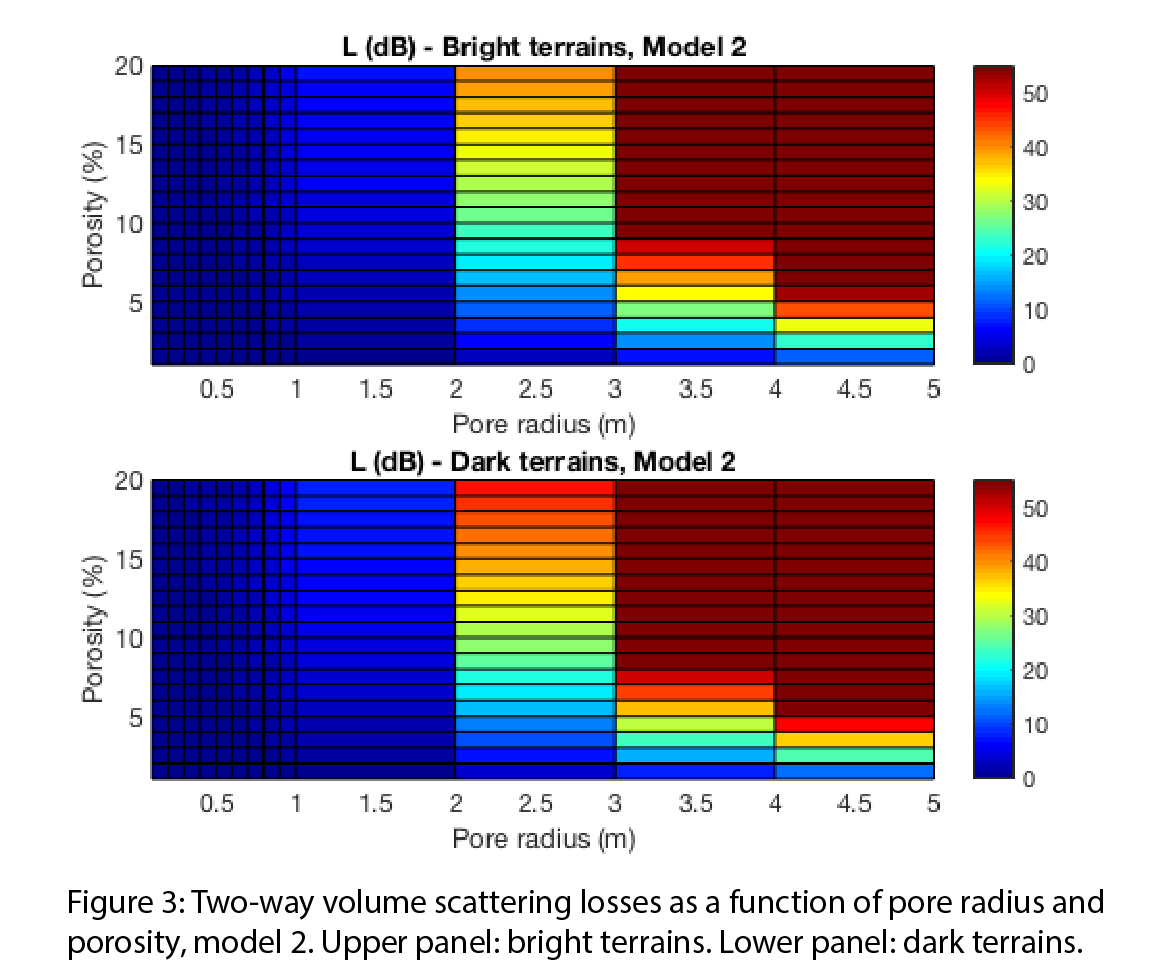
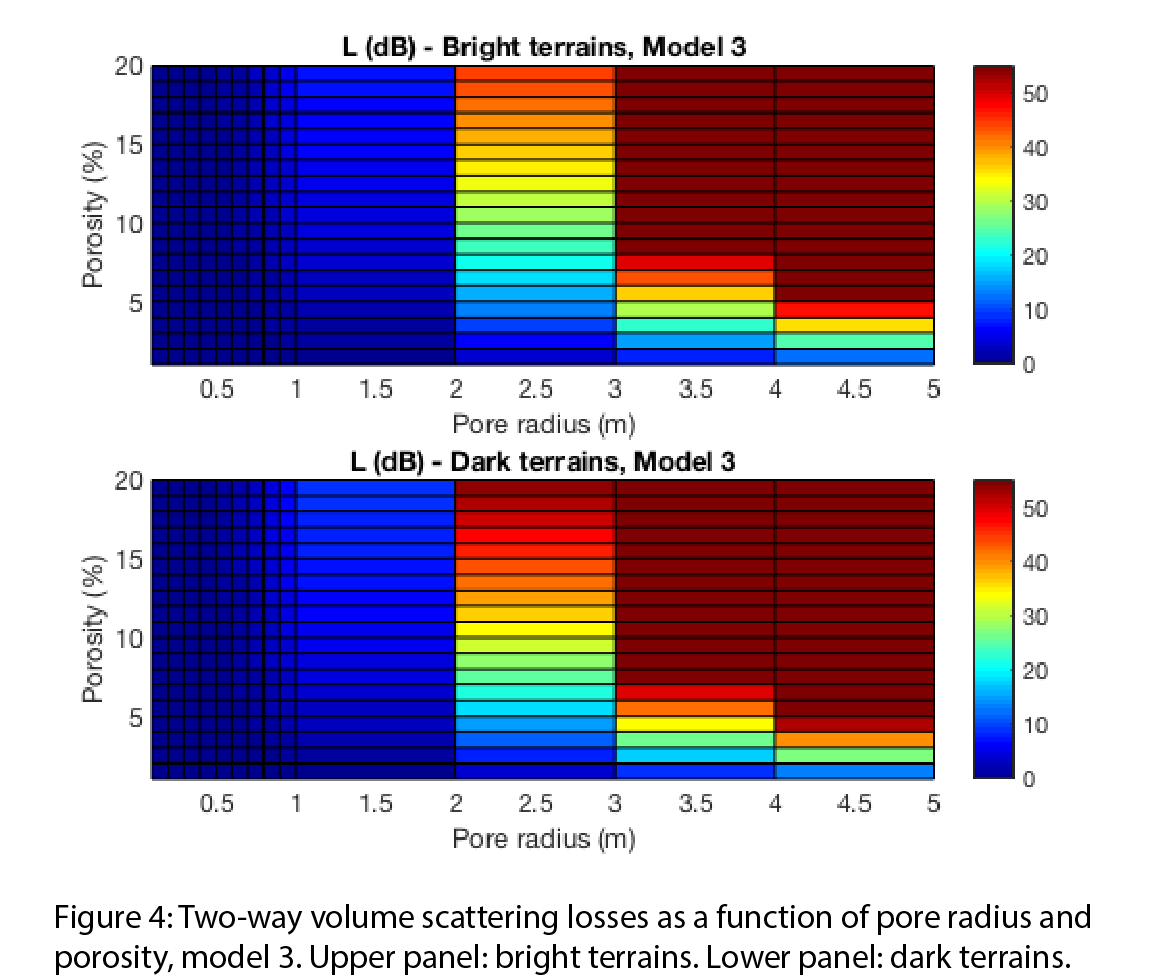
Conclusions
We performed numerical simulations of radar sounding of Ganymede, considering different dielectric models for silicate inclusions in the ice. In the case of a high-attenuation silicate, the attenuation is significantly higher with respect to the other two cases, but the radar sounding of the first kilometers will not be affected. Regarding the volume scattering losses, these will be significative only for pore radius bigger than 2.5 m, and such a value can be reasonably discarded. In such conditions we expect no significant obstacles for the radar sounding of Ganymede.
Acknowledgments
The Authors gratefully acknowledge financial support from the Italian Space Agency through Contract ASI-INAF 2018-25-HH.0.
References
Berquin Y. et al., 2013, Planet. Space Sci., 77, 40-44.
Bruzzone L. et al., 2013, IEEE International Geoscience and Remote Sensing Symposium, 3907-3910.
Di Paolo F. et al., 2014, 15th International Conference on Ground Penetrating Radar, 362- 366.
Di Paolo F. et al., 2016, IEEE J. Sel. Topics Appl. Earth Observ. Remote Sens., 10(1), 118-129.
Di Paolo F. et al., 2018, 5th IEEE International Workshop of Metrology for AeroSpace, 2422-2425.
Heggy E. et al., 2017, Icarus, 285, 237-251.
Ilyushin, Ya.A., 2014, Planet. Space Sci., 92, 121-126.
Kawada, S., 1978, J. Phys. Soc. Jpn., 44(6), 1881-1886.
Sihvola, A. H., 1999, Iet, 47.
Ulaby F. T. et. al., 1986, Addison-Wesle, 436 pp.
How to cite: Di Paolo, F., Lauro, S. E., Cosciotti, B., Mattei, E., and Pettinelli, E.: Radar sounding of Ganymede: Attenuation and volume scattering losses estimation, Europlanet Science Congress 2020, online, 21 Sep–9 Oct 2020, EPSC2020-291, https://doi.org/10.5194/epsc2020-291, 2020.
Radio Occultation is a very powerful technique to probe a planetary atmosphere, in providing vertical density profiles of the neutral atmosphere and ionosphere. The standard method uses a radio link at S and/or X band between a spacecraft and an Earth ground station. At Mars, such measurements are conducted since the 60s. The three most recent data sets are from MGS (1998-2006), Mars Express (since 2004) and MAVEN (since 2016). Taking advantage of two European spacecraft in orbit around Mars, the European Space Agency is currently preparing an experiment that consists of mutual radio occultations between Mars Express and the ExoMars Trace Gas Orbiter. Both spacecraft use UHF transceivers that are included primarily for communication between landers on the surface of Mars and the spacecraft, where the spacecraft act as relay orbiters to pass the data from the landers on to Earth. Therefore, these mutual occultations will be performed in the UHF range (centered around a frequency of 400 MHz). The feasibility of this technique on UHF was demonstrated between the NASA Mars Odyssey and Mars Reconnaissance Orbiters [Ao et al., 2015].
In this presentation, the advantages and challenges of this technique over the traditional spacecraft to Earth occultation measurements, the plans for conducting these experiments with Mars Express and the Trace Gas Orbiter, and the envisaged data processing technique will be briefly reviewed.
Before the data becomes available, and in order to prepare the data processing, a simulation-based strategy has been adopted to implement an algorithm able to retrieve vertical electron density profiles from Doppler shift measurements. More specifically, as a first step, simulated spacecraft orbits are calculated and a Chapman function is used to obtain the electron density of the Martian ionosphere. Subsequently, a numerical 3D ray-tracing algorithm [Kashcheyev et al., 2012] is applied to compute ray trajectories in the presence of the ionosphere and the relevant Doppler shift time series corresponding to the simulated radio occultation event. Then, assuming a spherical symmetry [Fjeldbo et al., 1971] for the ionosphere electron density, the (excess) Doppler data are converted to bending angles and impact parameters. Finally, the bending angle profile is inverted (through Abel integral) to a vertical refractivity profile, which, in turn, provides information about the ionospheric electron density.
For completeness, the simulation described above has been carried out with an exponential refractivity function defining the neutral atmosphere alone and with both the Chapman and the exponential refractivity functions to simulate the whole atmosphere of Mars.
The first results obtained by means of the mentioned approaches will be presented, with particular focus on the retrieval of the ionospheric electron density profiles.
References
Ao, C. O., C. D. Edwards Jr., D. S. Kahan, X. Pi, S. W. Asmar, and A. J. Mannucci (2015), A first demonstration of Mars crosslink occultation measurements, Radio Sci., 50, 997–1007, doi:10.1002/2015RS005750.
Fjeldbo, G., A. J. Kliore, and V. R. Eshleman (1971), The neutral atmosphere of Venus as studied with the Mariner V radio occultation experiments, Astron. J., 76, 123–140.
Kashcheyev, A., B. Nava, and S. M. Radicella (2012), Estimation of higher-order ionospheric errors in GNSS positioning using a realistic 3-D electron density model, Radio Sci., 47, RS4008, doi:10.1029/2011RS004976
How to cite: Nava, B., Kashcheyev, A., Migoya-Orue, Y., Radicella, S. M., Parrott, J., Sánchez-Cano, B., Witasse, O., Svedhem, H., Titov, D., and Ao, C. O.: Mutual radio occultation experiment between ExoMars Trace Gas Orbiter and Mars Express: feasibility study and preparation for the data analysis, Europlanet Science Congress 2020, online, 21 Sep–9 Oct 2020, EPSC2020-299, https://doi.org/10.5194/epsc2020-299, 2020.
Recent remote sensing missions have established the presence of water-ice in permanently shadowed regions (PSRs) located at the lunar poles, offering the possibility of a source of material for future In Situ Resource Utilisation (ISRU). ISRU offers the opportunity to make use of locally available resources and to potentially reduce the costs associated with transporting materials such as water, hydrogen and oxygen to the lunar surface. The LUVMI-X rover, is an innovative, low mass, mobile robotic payload designed specifically for operations at Polar regions of the Moon with a range of 5 km from its lander and will offer the opportunity to perform volatile prospecting for potential lunar resources. We discuss the LUVMI Volatiles Sampler (VS) and Volatiles Analyser (VA), known collectively as the Lunar Volatiles Scout (LVS) instrument, that has been developed and validated up to technology readiness level (TRL) 5-6.
The aim of the LUVMI mobile platform [1] is a traverse of up to a distance of 5 km from the landing site, and to perform prospecting for volatiles in the top 10 to 20 cm of the lunar regolith. The rover is designed around an aluminum structural frame with a four wheel drive train in a rocker-bogie configuration and is capable of driving on slopes of up to 20 degrees. Figure 1 shows the LUVMI prototype during mobility testing in 2018).
Figure 1 The LUVMI prototype rover during mobility and nighttime operational testing in December 2018
The Lunar Volatiles Scout (LVS) is is a small, low mass instrumented drill designed for the detection and analysis of volatiles in lunar regolith. It consists of the Volatiles Sampler (VS) and the Volatiles Analyser (VA). The VS is a 15 cm core drill that can heat samples in-situ in the ground. The (VA) is a miniature ion trap mass spectrometer (ITMS) with a mass range (m/z) between 10 and 150 for gas analysis (Figure 2). The LVS assembly has a combined mass of less than 2 kg [2].
Figure 2 An image of the prototype LUVMI VA ion trap instrument
To enable evaluation and characterization in the laboratory a facility was developed that would provide the following:
- Environmental (vacuum and thermal) conditions similar to the lunar environment
- Provision to provide reference gases to allow calibration of the instrument.
- Thermal control of regolith simulant material during volatile extraction experiments
A sample container (Figure 3) was manufacuted with four fins protruding into the interior to facilitate uniform sample cooling of NU-LHT-2M simulant materials. Cooling was achieved via a copper coil that was silver soldered to the sample container and passed through the walls of the vacuum chamber via a gas feed-through. A computer controlled liquid nitrogen (LN) valve was used to automatically control the flow of LN into the sample cooling coil. To reduce radiative losses from the sample container and the cooling coils all surfaces are wrapped with multi-layer insulating material. Temperature control of the sample from room temperature to -140˚C, down to a pressure of 2×10-5 mbar was achieved.
Figure 3 Showing the NU-LHT-2M sample loaded into the sample container prior to a volatiles extraction experiment
Regolith simulant samples were prepared and impregnated with 0.5, 1, 2% and 5% water content (by mass) to mimic volatile content that is consistent with the L-CROSS observations, where concentrations in the region of 5.6 ± 2.9% by mass were reported. The water impregnated samples were transferred to the sample container in the vacuum system, which was then evacuated before volatile extraction by insertion of the heated probe and measurements of the volatiles by ITMS.
The ion signal at m/z 18 was used as an indicator for the presence of water. The extraction experiments were operated for 1500 s for each of the four regolith samples. Following insertion of the heated probe into the sample, the intensity of signal measured by the ITMS was observed to slowly increase as water is liberated from the sample (corresponding to a pressure rise of 2×10-5 to 9×10-5 mbar before falling back to the 1×10-5 mbar ). As would be expected, with increasing water content the peak intensity of the ion signal at m/z 18 increases. A summary of the results from volatile extractions from samples containing 0.5,1,2 and 5% is shown in Figure 4.
Figure 4 Summary of the water extraction experiments from samples containing 0.5, 1 2 and 5% water by mass
The main objective of the VS and VA development was to provide a tool to address the LEAG VSAT priority measurement to determine the variability of volatiles distribution. The presented instrument is able to sample lunar regolith up to a depth of 20 cm, i.e. a depth at which water should be stable over wide areas of the polar regions (even in non PSRs). The ability of VS to extract volatiles and VA to identify the species present was demonstrated in a representative environment during the characterization campaign, which characterizes it with a TRL of 5-6, making it valid for near term scientific and ISRU volatile characterization missions.
This project is co-funded by the Horizon 2020 Framework Programme of the European Union, grant agreement 727220 and 822018
[1] J. Gancet et al (2019) IAC-19-A3.2C.6.
[2] J. Biswas et al. (2020) Planetary and Space Science., 182.
How to cite: Sheridan, S., Barber, S., Biswas, J., Regaanaz, M., and Richter, L.: Characterisation of the LUVMI Lunar Volatiles Scout Instrument, Europlanet Science Congress 2020, online, 21 Sep–9 Oct 2020, EPSC2020-900, https://doi.org/10.5194/epsc2020-900, 2020.
Please decide on your access
Please use the buttons below to download the presentation materials or to visit the external website where the presentation is linked. Regarding the external link, please note that Copernicus Meetings cannot accept any liability for the content and the website you will visit.
Forward to presentation link
You are going to open an external link to the presentation as indicated by the authors. Copernicus Meetings cannot accept any liability for the content and the website you will visit.
We are sorry, but presentations are only available for users who registered for the conference. Thank you.