Introduction
An invaluable source of information about the present interior structure of Venus is the measurement of surface deflections and disturbances to the planet's gravity field caused by tidal deformation. The planet is mainly loaded by the semidiurnal solar tide that probes the interior at the angular frequency ω ≈ 1.25×10-6 rad s-1 [e.g., 1]. The tidal response is then determined by the rheological properties of the body.
In this work, we combine interior structure and tidal modeling of Venus to compute and compare measurable quantities, namely the tidal Love numbers k2, the moment of inertia factor MoIF, and the tidal quality factor Q. Our predictions can be tested with future measurements, should current Venus mission proposals (e.g., Veritas and EnVision) be selected.
Interior structure and thermal state models
In order to calculate the tidal parameters (k2 and Q), we first generate 1-D interior structure models of Venus. Our method is similar to that of [2] in which the interior is subdivided into chemically separated layers: an iron core, a rocky mantle, and a basaltic crust.
In addition, steady-state temperature profiles are calculated by applying a mixing-length approach [3], and we vary poorly constrained parameters (crustal density and thickness, iron content in the mantle, reference viscosity of mantle and core, surface and core-mantle heat fluxes).
Tidal deformation models
Tidal deformation modeling is based on the normal mode theory for a radially-stratified nonrotating incompressible viscoelastic sphere [e.g., 4]. In this approach, the deformations, tractions, and additional potential in the planet are expanded into spherical harmonics and the governing equations for the viscoelastic continuum are solved analytically. The resulting deformation state is then described by parametric functions, the parameters of which can be obtained from the boundary conditions.
We compute the potential Love numbers by using two different techniques. In the first approach, the radial profiles of the deformations, tractions, and additional potential are computed by using a matrix propagation method [e.g., 5, 6]. In the second approach, we seek directly the parameters of the aforementioned parametric functions [7].
We use the well-known Maxwell model for the core and adopt an Andrade model to compute the response of the Venusian mantle and crust [8, 9]. In contrast to the Maxwell model, the Andrade rheology accounts not only for the instantaneous elastic deformation and the viscous (steady-state) creep but also for the transient effects governed by the Andrade hereditary terms.
Results
(a) Comparison of Love numbers
We select representative interior structure models and compute the tidal parameters according to the two methods described above. Both methods provide the same results for various sets of input parameters. Furthermore, we compare our results with published data from [1]. To this end, we used the same interior temperature profiles, core size, and viscosity as in [1], and employed two end-member compositions (i.e., BVSP(81)-Ve1 with a low and BVSP(81)-Ve4 with a high iron content in the Venusian mantle) similar to their models V1 and V4. Our results in Fig. 1, calculated with the tidal model of [7], are in close agreement with the data presented in Fig. 4 of [1].
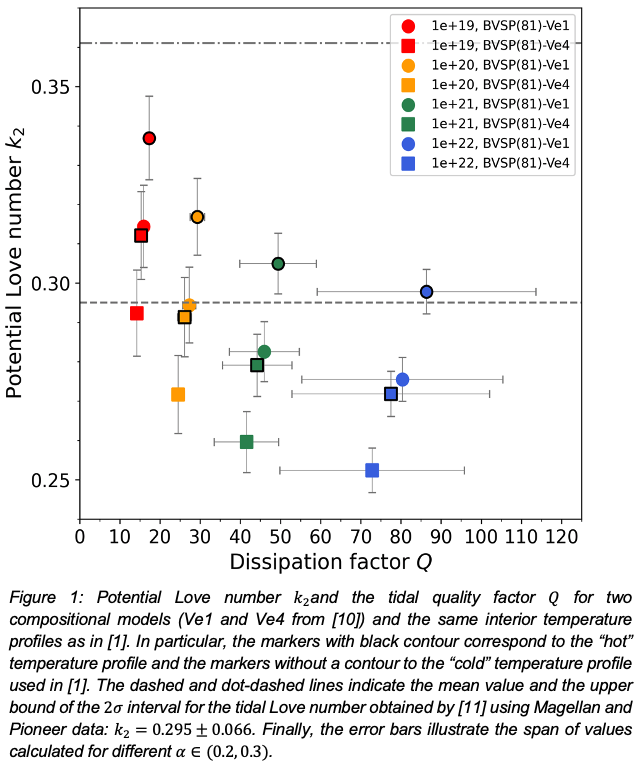
(b) Effect of mantle composition
We observe two distinct ranges of MoIF according to the bulk composition used in our interior structure models (Fig. 2). All models corresponding to the mantle mineralogy with a negligible low iron content [BVSP(81)-Ve1] are found at significantly lower MoIF than their iron-rich [BVSP(81)-Ve4] counterparts. Furthermore, it can be seen that an iron-rich mantle leads to smaller core sizes when comparing the two compositional models.
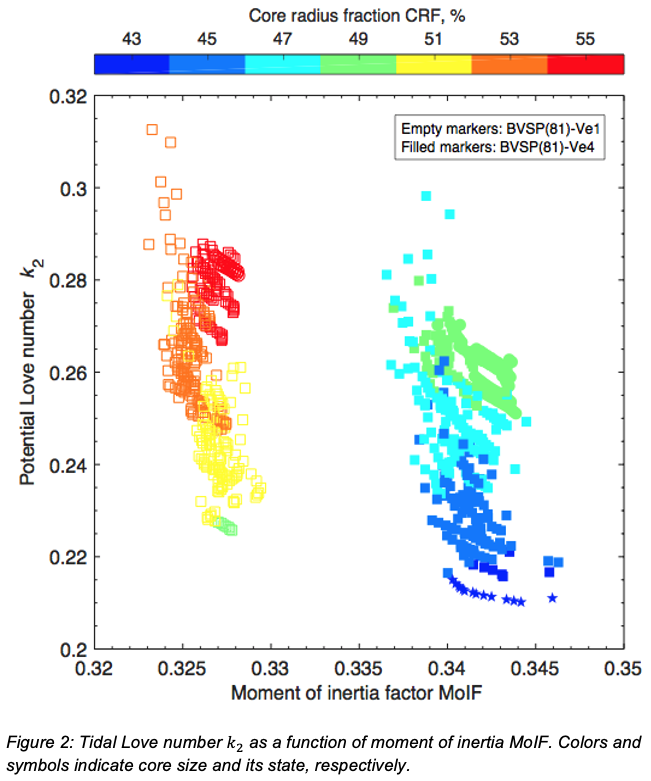
Combining the MoIF and the potential Love number k2 delivers additional information about the state and size of the core. In Figure 2, the models with a completely solid core (stars) have the smallest core size and thus the lowest k2, whereas the models with a completely liquid core (circles) have in general large core sizes and thus high k2. In between these two end-members, we find the models with solid inner and liquid outer cores (squares). These models usually have medium-sized cores and intermediate potential Love numbers k2. However, the highest k2 value is obtained for models with partially liquid iron cores. Their much hotter counterparts with completely liquid cores, which would lead to even higher k2, contain a fully molten mantle layer, and have been excluded here. Thus, the determination of the state and size of the core could be challenging for high potential Love numbers k2 due to a model degeneracy from input parameters.
Conclusions
In this study, we have presented a comparison between two tidal deformation models [6,7] to compute Venusian tides. The results show a close agreement between the two tidal deformation models and the study of [1].
We have applied our interior models to investigate the effects of composition on the tidal parameters and moment of inertia factor. The results indicate two families of models with distinct MoIF that could be constrained by future measurements.
In the next step, we plan to combine the tidal deformation model with thermal evolution calculations to study the secular tidal evolution. To this end, we will couple the tidal model to 1-D parametrized [e.g., 12] and 2-D geodynamical [e.g., 13] models that treat the cooling history and melt production in the interior of Venus. Furthermore, our coupled tidal-thermal evolution model can be applied to study the interior of Venus-like extrasolar planets.
References
[1] Dumoulin, C. et al. (2017): JGR:Planets, 122.
[2] Sohl, F. & Spohn, T. (1997): JGR:Planets, 102(E1).
[3] Wagner, F. W. et al. (2019): GJI, 217(1).
[4] Sabadini, R. & Vermeersen, B. (2004): Springer.
[5] Segatz, M. et al. (1988): ICARUS, 75(2).
[6] Steinbrügge, G. et al. (2018): JGR:Planets, 123(10).
[7] Walterová, M. & Běhounková, M. (2020): ApJ, 900(1).
[8] Jackson, I. & Faul, U. H. (2010): PEPI, 183.
[9] Castillo‐Rogez, J. C. et al. (2011): JGR:Planets, 116(E9).
[10] Basaltic Volcanism Study Project (1981): Pergamon Press.
[11] Konopliv, A. S. & Yoder, C. F. (1996): GJI, 23(14).
[12] Tosi, N. et al. (2017): A&A, 605.
[13] Plesa, A.-C. & Breuer, D. (2021): LPSC, No. 2548.