Introduction: Because of early recurring impacts and later impact gardening, lunar crust surface turned into a layer of fragmented, variably shocked and occasionally melted impact breccias [Heiken et al., 199; White et al., 2020].
One of the most severely affected group of rocks found on the lunar surface is the Mg-suite group. These are Mg-rich, primitive, plutonic to hypabyssal coarse-grained rocks which texture and bulk composition reflect magmatic accumulation of mineral phases [Heiken et al., 1991; White et al., 2020; Shearer et al., 2015; Černok et al., 2020].
Whether the Mg-suite rocks formed by partial melting of the lunar mantle or they are impact-related is still debated [Taylor et al., 1993]. In the latter case, their origin could be related to the melting of early lunar crust and mantle caused by frequent hypervelocity impacts [Jolliff et al., 2006].
Some of their minerals, like Fe-Ni metals and sulfides, reflect mixing and melting of impactor(s) and target rocks and the proportion of impactor vs. target contributions [Tang et al., 2023; Day et al., 2020]. For example, a high Ni/Co ratio in Fe-Ni metal grains can be the consequence of the addition of either iron or chondritic impactors. Furthermore, those same minerals are extremely sensitive to processes that happened in Moon’s interior, such as fractional crystallization [Day et al., 2020]. For example, Ni/Co ratio decreases when mineral phases like pyroxene and plagioclase crystallize together because of the different compatibility of Ni and Co in both solid and melt.
For these reasons, those minerals cannot only be considered important means to investigate lunar rocks origin, but also lunar evolution processes [Day et al., 2020]. In this study we concentrate on defining the mineral chemistry of Fe-Ni metal and sulfide grains in a set of variably shocked breccias from three different Apollo missions (15, 16 and 17).
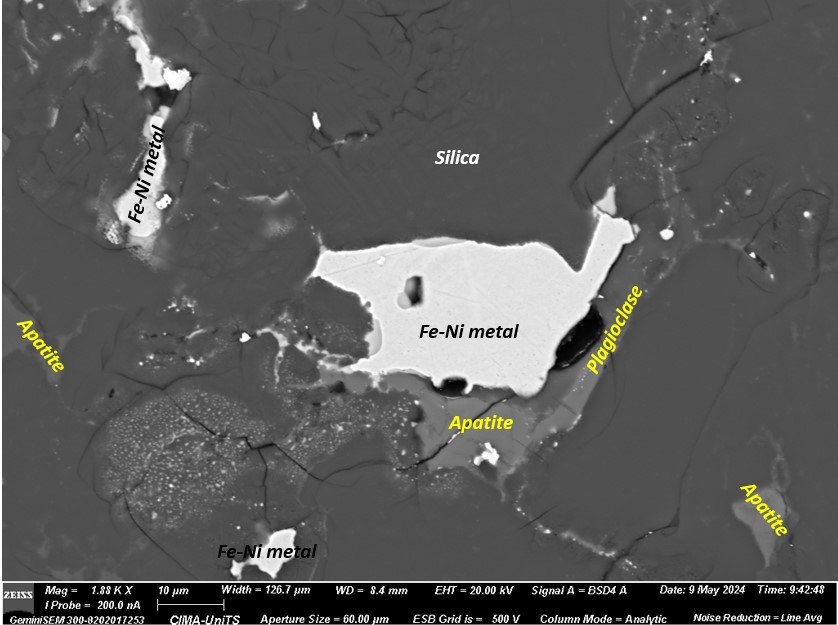
Figure 1. Fe-Ni metal grain in thin section 78235,38, BSE image
Samples: Three different shocked breccia samples were selected from Apollo 15, 16 and 17 collections: shocked norite 78235 (thin sections 78235,38 and 78235,51), mainly consisting of glass veins and cumulus and partially fractured orthopyroxene and plagioclase, with much of the plagioclase converted into maskelynite [Meyer, 2010]; dimict breccia with shocked norite 15455 (thin sections 15455,27 and 15455,28), primarily made of fragmented orthopyroxene and plagioclase in a KREEP-rich, fine-grained igneous-textured groundmass containing plagioclase, olivine and pink spinel clasts [Meyer, 2010]; feldspathic polymict breccia 67915, showing two-main polymict lithologies, one white and one grey, containing heterogeneous lithic clasts cemented in shock-melted glass (thin sections 67915,76 and 67915,84) [Meyer, 2010].
Methods: Non-destructive chemical analysis were performed at the Institute of Geological Sciences at the Freie Universität Berlin (Germany) using a JEOL JXA 8200 Superprobe on minerals selected with the help of QEMSCAN maps and BSE images. In both metals and sulfides we analyzed the concentration of siderophile (Fe, Ni, Co, Mn), chalcophile (S, Zn, Cu) and some lithophile elements (such as Ca, Mg, Si, Cr, P, used to evaluate possible interference by surrounding silicates). For all the elements detection limits lie between 100 and 200 ppm, while the beam size was usually 1 μm.
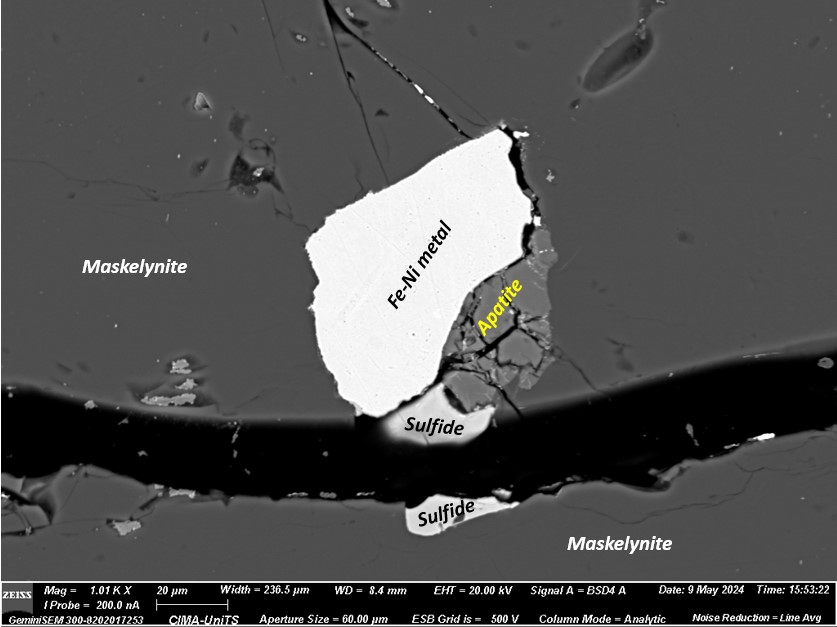
Figure 2. Sulfide and Fe-Ni metal grains in thin section 78235,51, BSE image
Initial results: In total, more than 150 grains were analyzed in four different thin sections, but most of them were very small (<1 µm), thus not suitable for chemical analyses. In sample 78235 three metal grains found in glass veins from 78235,51 show higher Ni/Co (from ~10.5 to ~20) and lower Fe/Ni (from ~3.6 to ~10.2) ratios. Remaining metal grains found in glass veins or in cumulus plagioclase (maskelynite) and/or pyroxene from the host norite show lower Ni/Co (from ~0.8 to ~0.9) and higher Fe/Ni (from ~35 to ~45) ratios. Similarly, sulfide grains generally plot within a restricted Ni/Co (from ~0.3 to ~0.5) and Fe/Ni (from ~1300 to ~2100) range of values, but grains from maskelynite tend to cover a wider range (Ni/Co from ~0.1 to ~1, Fe/Ni from ~52.5 to >6700). Different is in 78235,38, where all metal grains plot within a small range of values (Ni/Co from ~0.7 to ~0.8, Fe/Ni from ~41.8 to ~48.7), while sulfide grains generally show a higher Ni/Co (from ~1.4 to ~3.1) and Fe/Ni (from ~1600 to >6800).
In sample 15455 most of the selected grains were found in the impact melt, but are usually <1 μm. The larger, albeit rare, grains (larger than 7 x 7 μm) were found in plagioclase and orthopyroxene clasts. In sample 15455, as in 78235,51, metal grains in the groundmass show variable Ni/Co (from ~0.8 to ~29.3) and Fe/Ni (from ~6.4 to ~80.2) compared to the ones found in plagioclase (Ni/Co from ~0.8 to ~2.1, Fe/Ni from ~5.1 to ~15) and orthopyroxene (Ni/Co from ~0.6 to ~0.8, Fe/Ni from ~18.1 to ~57.7). Sulfide grains show slightly higher Ni/Co (from ~0.6 to ~15 for pyroxenes, from ~2.3 to 5 for plagioclase) and lower Fe/Ni (from ~480 to >4700 for pyroxenes, from ~9.3 to >4700 for plagioclase) compared to sulfides in 78235.
In sample 67915 chemical analysis are yet to be performed.
Preliminary conclusions: The calculated Ni/Co and Fe/Ni from the investigated Fe-Ni metals in differently shocked Apollo Mg-suite samples indicate that at least some are of possible impact-related origin. Fe-Ni metal grains within glass veins in sample 78235 and matrix in sample 15455 usually show Ni/Co ~20, compatible with possible iron meteorite contamination, as shown by Day et al (2020) and McCallum and Mathez (1975). FeNi metal grains belonging to other mineral assemblages have lower Ni/Co, usually ≤1, which is more compatible to an endogenous origin [Day et al., 2020]. This is also evident in sulfides, were a Ni/Co ratio that varies between ~0.3 and ~5 suggest a more endogenous origin. Fe/Ni values in Fe-Fe-Ni metals are mostly compatible with kamacite metal (>92% Fe, <7% Ni) [Day et al., 2020].