Photochemical hazes are expected to be present in exoplanet atmospheres with quantities spanning a large range of values, from quasi-non-detectable traces in clear atmospheres, to large amounts dramatically modifying their environment. Our purpose is to determine the properties of these haze particles in hot- Jupiters’ atmosphere.
We first calculate thermochemical abundances (Gordon & McBride, 1994) and haze distributions from a microphysics model (Lavvas & Koskinen, 2017), using clear-atmosphere temperature profiles (Sing et al., 2016). This allows to derive the theoretical spectrum that can be be confronted to the observations to determine the best couple of parameters defining the haze production. These best-fitting parameters are then used in a disequilibrium chemistry (Lavvas et al., 2014) and microphysics model, providing a more detailed picture of the atmosphere. The calculated chemical and haze distributions allow for the evaluation of a new temperature profile (Lavvas & Arfaux, 2021) for each planet which can be used in the disequilibrium chemistry model. This process is repeated until the model is converged providing a self-consistent picture of the atmosphere.
Disequilibrium chemistry accounts for transport of species through the atmosphere as well as their interactions with the radiation field. The implications of these effects are revealed by comparing the thermochemical equilibrium abundances with those derived by the disequilibrium model. Chemical distributions basically show larger mixing ratios between 1e-2 and 1e-6 bar compared to thermochemical equilibrium and lower mixing ratios above 1e-6 bar altitude related to the destruction of molecules through photolysis (Fig. 1). These modifications of the chemical composition do not have significant impact on the calculated transit spectra (Fig. 2) considering the resolution and precision of the observations and the still small abundances of the modified species. Water, that has a major signature on the transit spectra is modified above 1e-6bar, in a region that is not affecting by the observations. However, the disequilibrium chemistry allows for the calculation of the mass flux from the photolysis of species considered as haze precursors, thus providing additional constraints to assess the realisticity of the haze production parameters retrieved from thermochemical equilibrium.
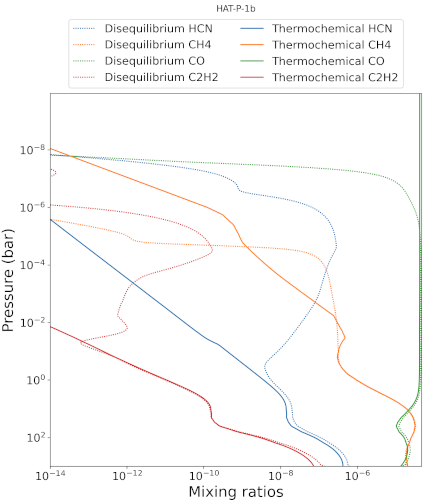
Fig.1: Haze precursors mixing ratio comparison between thermochemical and disequilibrium abundances.
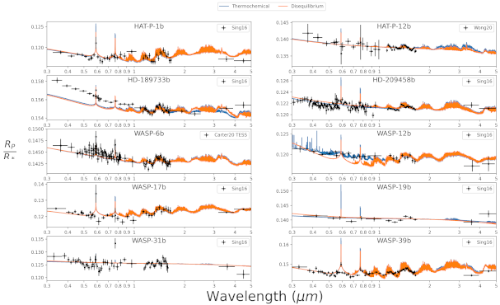
Figure 2: Transit spectra obtained from thermochemical equilibrium (blue line) and disequilibrium chemistry (orange line) compared to observations (black crosses) from Sing et al. (2016); Wong et al. (2020); Carter et al. (2020)
Disequilibrium composition gases and haze opacities have major impact on the temperature profile (Lavvas & Arfaux, 2021) which, in turn, has a large influence on the chemical composition and haze growth. These feedback effects are investigated through a self-consistent model coupling temperature, haze and chemical profiles calculation. Results show a clear trend for the increase of temperature under the influence of haze heating (Fig. 3), which has further ramifications on chemistry and haze profiles.

Figure 3: Self-consistently derived temperature profiles (dotted lines) and initial haze-free temperature profiles (solid line) from Sing et al. (2016) for HD- 189733b (left panel) and WASP-6b (right panel). The horizontal black lines corresponds to the radiative/convective boundaries.
These modifications in the atmospheric chemical and microphysics composition have a major impact on the transit spectra, with the most important feature being the increase of the atmospheric scale height due to the higher temperatures, thus yielding a larger transit depth. This last effect can thus enhance the steepness of the UV-visible slope observed in the spectra (Fig. 4) and decreases the requirement for a strong haze mass flux. These results demonstrate the need for a self-consistent description of haze interaction with the atmosphere.

Figure 4: Disequilibrium (red line) and self-consistent (blue line) theoretical spectra compared to observations (black crosses).
References
Figure 4: Disequilibrium (red line) and self-consistent (blue line) theoretical spectra compared to observations (black crosses).
Carter, A. L., Nikolov, N., Sing, D. K., Alam, M. K., Goyal, J. M., Mikal-Evans, T., Wakeford, H. R., Henry, G. W., Morrell, S., Lòpez-Morales, M., Smalley, B., Lavvas, P., Barstow, J. K., Garcìa Mun ̃oz, A., Gibson, N. P., & Wilson, P. A. (2020). Detection of Na, K, and H2O in the hazy atmosphere of WASP-6b. Monthly Notices of the Royal Astronomical Society, 494(4), 5449–5472.
Gordon, S., & McBride, B. J. (1994). Computer Program for Calculation of Complex Chemical Equilibrium Compositions and Applications I. Analysis. National Aeronautics and Space Administration (NASA).
Lavvas, P., & Arfaux, A. (2021). Impact of photochemical hazes and gases on exoplanet atmospheric thermal structure. Monthly Notices of the Royal Astronomical Society, 502(4), 5643–5657.
Lavvas, P., & Koskinen, T. (2017). Aerosol properties of the atmospheres of extrasolar giant planets. The Astronomical Journal, 847.
Lavvas, P., Koskinen, T., & Yelle, R. V. (2014). Electron densities and alkali atoms in exoplanet atmospheres. arXiv e-prints, (p. arXiv:1410.8102).
Sing, D. K., Fortney, J. J., Nikolov, N., Wakeford, H. R., Kataria, T., Evans, T. M., Aigrain, S., Ballester, G. E., Burrows, A. S., Deming, D., Désert, J.-M., Gibson, N. P., Henry, G. W., Huitson, C. M., Knutson, H. A., Lecavelier Des Etangs, A., Pont, F., Showman, A. P., Vidal-Madjar, A., Williamson, M. H., & Wilson, P. A. (2016). A continuum from clear to cloudy hot-Jupiter exoplanets without primordial water depletion. Nature, 529.
Wong, I., Benneke, B., Gao, P., Knutson, H. A., Chachan, Y., Henry, G. W., Deming, D., Kataria, T., Lee, G. K. H., Nikolov, N., Sing, D. K., Ballester, G. E., Baskin, N. J., Wakeford, H. R., & Williamson, M. H. (2020). Optical to near-infrared transmission spectrum of the warm sub-saturn hat-p-12b. The Astronomical Journal, 159(5).