Abstract
The WISDOM ground penetrating radar aboard the Rosalind Franklin rover is waiting for its intended launch in 2028 within the ExoMars mission. It will search for Water, Ice, and Subsurface Deposit On Mars (WISDOM) to enhance the knowledge of Oxia Planum on Mars. Meanwhile, the WISDOM team is improving the signal processing, since in general the microwave scattering in the sensing channel is a very complex phenomenon [1]. It is mainly determined by the property of transceiver system (radar), of the wireless channel in the random media and the property of the targets to explore. Regarding the target property, it can be divided into two main factors, namely the geometry/shape and the material properties (frequency-dependent relative permittivity). In practice however, due to the high complexity and sensibility of the random scattering signal tracking and separating of these responses is a challenging task by pre-calibration such that it can deteriorate e.g. the subspace image analysis. With radar systems such as WISDOM, which have a distance of up to several wavelengths between the antenna and the ground, there is furthermore an influence from the environment on the radar coupling, which results in an unknown signal. A reasonable tracking/estimation, especially on-site calibration, of these random signal plays then a major role for the radar technologies in practice and will be outlined in this paper.
1 Introduction
In this paper, we will mainly focus on the random scattering signal analysis for WISDOM and similar GPR-like sensing systems, where the distance between radar system and targets could be very short (close to the antenna near-field). Such that there can be severe signal inter-action between radar and targets.
As a result, both the calibrated radar response and the characteristic response of the target will be distorted and result in unknown signal. In other words, a pre-calibration for radar system and targets in this case will fail. For that, we suggest an on-site coordinate system for those applications.
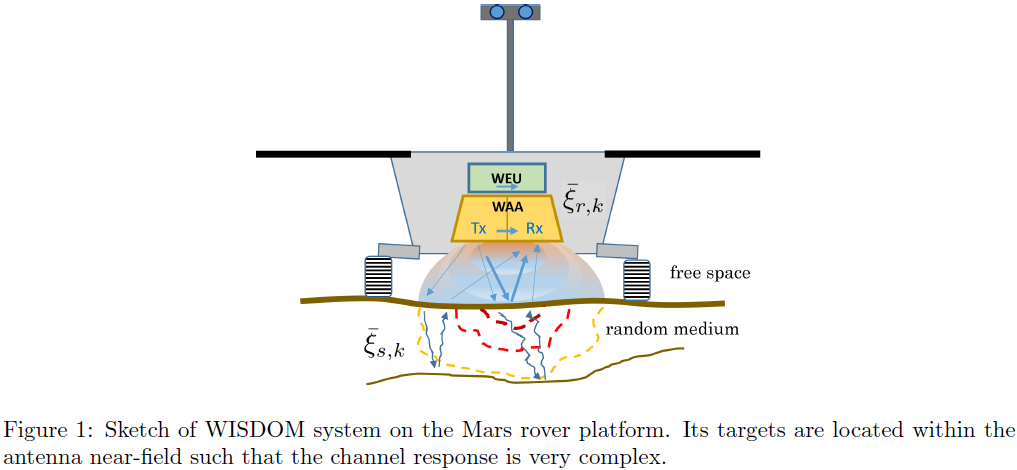
Regarding concrete applications, it can be summarized (but not limited) as follows:
- on-site calibration
- higher resolution imaging
- geological feature analysis.
For the purpose above, we introduce the kernel function ξ, which describes each independent channel spectral response, for signal modelling.
The whole received signal then consists of a sum of all convolutions between different kernel functions and their channel impulse responses h. Regarding j-th measured data, that is

However, both kernel functions and their channel impulse responses are unknown in general, due to the quasi near-field effect.
2 On-Site Calibration
The on-site calibration for WISDOM is mainly focusing on the free-space response tracking. This free-space is basically the radar initial working circumstance which is influenced by e.g. the rover platform and Mars ground. This means, every new measured data have always its own coordinate system.
The on-site coordinate system will be extracted by the kernel decomposition, particularly of the strong reflected signal, which are usually dominated by the surface reflected signal. The corresponding coordinate system is equivalent as an on-site calibration in terms of free-space circumstance.
Given multiple measurements, by exploring the coherent property among different data, the calibrated kernels and their channel impulse responses can be summarized as

where Δ is the tracking algorithm. This autonomous calibration is important especially for subsurface signal analysis in terms of e.g. subspace imaging and subsurface scattering dictionary construction. Since all subsurface signal can be considered as some kinds of deviation of the surface signal.
3 Higher Resolution Imaging
High spatial resolution for radar imaging is always an important issue which have been discussed and there are different ways for handling this problem. Generally, the radar image resolution (matched filter based) is limited by the system effective signal bandwidth, which is however finite in practice. An approach to extend the bandwidth by extrapolation for improving range resolution is given in [2].
By considering our model in Eq. 1, the mentioned limited spatial resolution is due to the bandwidth-dependent pulse width of kernel function ξi, while its channel impulse response hi is a sequence of Dirac pulses and exhibits unlimited spatial resolution. Therefore, instead of achieving larger bandwidth, we are trying to extract the channel impulse response alternatively. For pursuing hi, a deconvolution of the kernel function ξi is required, which in turn still depends on the on-site response ξi tracking. In other words, kernel tracking (KT) is a dual problem for higher resolution imaging. In practice, both BWE and KT are proposed for cross-validation.
An extended perspective for higher resolution imaging is a higher precise estimation of kernel function ξi, which plays a major role for the geological feature's analysis. Basically, the tracking algorithm in Eq. (2) is an iterative process where ξi and hiare alternatively estimated and tracked. A better estimation of one parameter can improve another parameter estimation. Thus, channel impulse response hi tracking is also a dual problem for kernel function estimation. Finally, higher resolution imaging can be considered as a joint estimation of ξi and hi.

4 Geological Features Analysis
Geological features analysis is basically the core of the WISDOM instrument, where the Mars surface and shallow subsurface properties will be explored. By the WISDOM radar system, these geological features are imprinted mainly over kernel functions ξi, such that kernel function tracking is geological features tracking as well. Certainly, so far we have just indicated this relationship between kernel function and geological properties and therefore cannot describe this relationship explicitly. Besides, this relationship can be distorted easily by all kinds of interference. Nevertheless, one can still present the geological features but in the relative manner, e.g. by unsupervised learning.
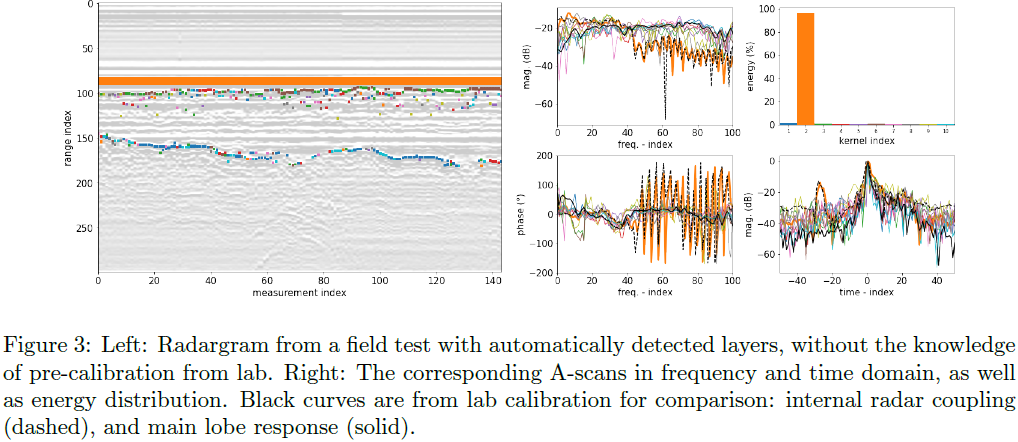
References
[1] A. Ishimaru "Wave Propagation and Scattering in Random Media", IEEE Press and Oxford University Press. ISBN 0-7803-3409-4, 1997.
[2] N. Oudart, V. Ciarletti, A. Le Gall, M. Mastrogiuseppe, Y. Hervé, W.-S. Benedix, D. Plettemeier, et al. “Range Resolution Enhancement of WISDOM/ExoMars Radar Soundings by the Bandwidth Extrapolation Technique: Validation and Application to Field Campaign Measurements.” Planetary and Space Science 197 (March 1, 2021): 105173. https://doi.org/10/ght9hk.