Introduction: The Package for Resource Observation and in-Situ Prospecting for Exploration, Commercial exploitation and Transportation (PROSPECT) is a payload in development by ESA for use at the lunar surface. Current development is for flight on the Russian-led Luna-Resource Lander (Luna 27) mission, which will target the south polar region of the Moon.
PROSPECT will perform an assessment of the volatile inventory in the near surface regolith (down to a depth of ~ 1 m), and elemental and isotopic analyses to determine the abundance and origin of any volatiles discovered [1] (Fig. 1).
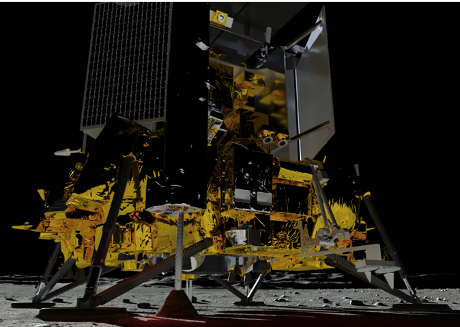
Credit: ESA/ROSCOSMOS/IKI/Lavochkin Association.
Figure 1: Graphical representation of PROSPECT onboard Luna 27 during surface operations.
In addition to the volatile studies, PROSPECT will perform a demonstration of In-Situ Resource Utilization techniques, extracting solar-wind implanted oxygen from lunar minerals. This demonstration will constitute potential science return from anywhere on the Moon, regardless of volatile content.
PROSPECT is comprised of the ProSEED drill module (including a permitivity sensor on the drill), and the ProSPA analytical laboratory, plus the Solids Inlet System (SIS) (Fig. 2).
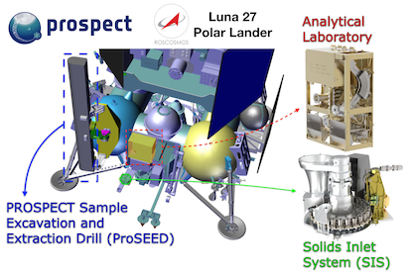
Credit: LDO / NPOL / IKI
Figure 2: Renderings of PROSPECT onboard Luna 27, including the ProSEED drill (left), and ProSPA (right). ProSPA comprises 1) the Solids Inlet System (lower right), with a camera assembly [2] and a carousel of ovens used for volatile extraction, and 2) the analytical laboratory (upper right) containing a gas processing system, and magnetic sector plus ion-trap mass spectrometers.
Development status and current activities: Phase C (detailed definition) began in December 2019. In parallel to the industrial activity, an associated plan of research has been formulated to guide ongoing development, build strategic scientific knowledge, and to prepare for payload operations.
Drill Testing. Tests of the ProSEED Development Model wer carried out in December 2019, including drilling into, and sampling from, well-characterized NU-LHT-2M simulant mixed with anorthosite inclusions of various plausible sizes [3, 4]. The main functionalities of the drill system were successfully demonstrated and required performances were achieved in these tests.
ProSPA Bench Development Model (BDM). The BDM of the ProSPA analytical lab at the Open University has been tested to demonstrate science performance against measurement requirements. Dedicated efforts recently focused on verification of evolved gas analysis (EGA) and demonstration of ISRU capabilities [5, 6], improving our understanding of our sensitivity to volatile abundance and possible contamination [7].
Volatile Preservation: Particular efforts have recently focused on understanding the capability of PROSPECT to sufficiently preserve the volatile content in regolith throughout the sampling-analysis chain for the range of expected volatile contents, e.g. [8]. Detailed modelling and experimental work is ongoing to better understand water sublimation rates in realistic lunar surface operational environments, regolith structures, and geometries [9], and to better constrain the potential effect on measured D/H of sublimation of lunar water ice [10]. Results from this will help ensure that even in a ‘hot operational case’, the original volatile inventory can be determined with sufficiently small uncertainties.
Sample analysis: In 2020, PROSPECT Science Team members successfully requested two samples of lunar regolith (2 g each) from the Apollo collections. Proposed experiments will investigate loss of water ice through sublimation and the effects that the bulk properties and the ice-regolith coupling have on the sublimation process.
ProSEED Imaging System: ProSEED includes an imaging system (Fig. 3) with LED illumination, which will be used to image the drilling operations, the build-up of the ‘cuttings pile’ as the drill descends, and the samples as they are transferred to the ProSPA laboratory for analyses. The Engineering Model of the imaging system is currently with the science team for testing, and is being fully characterized from a scientific perspective. This will include some dedicated testing to see how the performance of the imager and the LED illumination changes in response to increasing coverage by simulated lunar dust.
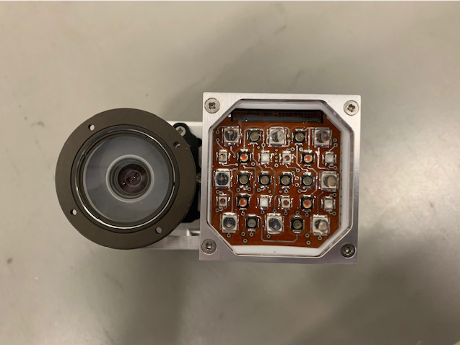
Credit: LDO / Kayser Italia
Figure 3: The PROSPECT Imaging System, with LED illumination.
Operational profiling: Work is currently underway to define operational priorities and preferred operational sequences. These are being discussed from a purely scientific perspective for now, and will later be fed into an operational database (Fig. 4) that will allow us to balance the spacecraft resources available against the desired scientific output. This work includes deciding on prospective drilling depths and the ProSPA analyses to be completed, depending on whether we would like to be looking at isotopic abundance, Evolved Gas Analyses, and/or ISRU experiments. There are many factors that need to be considered when defining these sequences, and some of these will be outlined in the presentation.

Figure 4: Operation spreadsheet for measurement prioritization.
PROSPECT Ion-Trap Mass Spectrometer (PITMS): A variant of the ProSPA ion-trap mass spectrometer has been selected for flight on the Astrobotic Peregrine-1 mission, which will fly to Lacus Mortis in 2021. PITMS will monitor the decay in the local exospheric pressure following landing, providing knowledge on lander-sourced contamination by scanning m/z 10 to 150 at 10 Hz, and time-integrating mass spectra in-situ to build S/N.
Summary: Development is now well underway for the PROSPECT package on Luna 27. This presentation will outline the development status, some science team activities, and the propespective scientific analyses to be undertaken once at the lunar surface.
References: [1] Trautner, R. et al., (2018) in Proc. Int. Astronaut. Congr. IAC, Vol. 2018-October. [2] Murray, N. J. et al. (2020) in Lunar Planet. Sci. Conf., LPI, Abs #. 1918. [3] Martin, D. J. P. and Duvet, L., (2019) in Lunar Planet. Sci. Conf., LPI, Abs #. 2663. [4] Hayne, P. O. et al., (2017) J. Geophys. Res. Planets 122 (12), 2371–2400. [5] Sargeant, H. M. et al., (2020) Planet. Space Sci. 180 (104751). [6] Sargeant, H. M. et al., (2020) in Lunar Planet. Sci. Conf. LPI, Abs #. 2058. [7] Abernethy, F. A. J. et al., (2020) Planet. Space Sci. 180 (104784). [8] King, O. et al., (2019) Planet. Space Sci. 104790. [9] Formisano, M. et al., (2019) Planet. Space Sci. 169. [10] Mortimer, J. et al., (2018) Planet. Space Sci. 158, 25–33.