Uranus and Neptune have a tropopause, stratosphere and a thermosphere inexplicably too hot. This problem is called "Energy Crisis" [1,2]. Radio occultation measurements [3,4] and UV stellar and solar measurements [5,6] by Voyager 2 revealed that their stratosphere reach values of 150 K and their thermosphere reach values of 800 K. Globally-averaged temperature observations [7,8] have also shown a warmer stratosphere than expected. Current radiative equilibrium models can’t explain the observed temperatures [9,10], one or several unknown heat sources are necessarily responsible of this warm atmosphere. Thus, the nature of this or these heat sources is still an open question.
To evaluate the role of several heat sources, we use the radiative-convective model developed by the Laboratoire de Météorologie Dynamique (LMD), previously used for the atmospheres of gas giant planets [11,12]. The model employs the correlated-k formalism to compute gaseous opacities from CH4, C2H2 and C2H6, opacities from collision-induced absorption (H2-H2, H2-He, H2-CH4, He-CH4 and CH4-CH4) assuming a H2 ortho/para fraction at equilibrium. Our model accounts for the internal heat flux, for multiple scattering and Rayleigh scattering. Cloud and aerosols physical properties (exctinction coefficient, single scattering albedo and asymmetry factor) are computed offline assuming Mie scattering, given a particle size distribution and optical constants. In the radiative-convective model, their optical depth is computed for each layer from several entry parameters: the total optical depth (at a reference wavelength), the boundary pressures (bottom and top of the layer), and an aerosol scale height [13].
Three hypotheses were explored separately to induce sufficient heating to warm the atmosphere. We varied the methane mole fraction profile, added an ad hoc aerosol layer, and imposed thermal conduction from the thermosphere.
Values of observed methane molar fraction in the uranian stratosphere being scattered [14], a sensitivity study was carried out by varying the methane homopause altitude and the stratospheric molar fraction. As expected, a high molar fraction makes it possible to heat the stratosphere and to obtain a tropopause altitude similar to observations. Nevertheless, all tests failed to fully heat the tropopause. This is also expected, as much less solar photons in the near infrared reach these levels.
Concerning thermal conduction from the thermosphere, the heating allows to obtain a temperature gradient in upper stratosphere similar to nominal temperature profile from [7]. However, simulated temperature profile is still colder (see figure 1).
Simulations showed that temperature profile of Uranus was very sensitive to the addition of an optically thin aerosols layer confined to a few levels in the stratosphere. To obtain a sufficiently important stratospheric heating, this layer requires that the absorption spectrum is relatively intense in ultraviolet and thermal infrared. This type of spectrum is similar to tholins, black carbon or a carbon-NH3 mixture [15]. The relatively dark spectrum of this layer also induces greenhouse effect in the tropopause.
In the case of Neptune, all hypothesis tested couldn’t warm stratosphere. Therefore, other heat sources are needed to explain observations, such as a dynamical forcing in stratosphere (...) and/or a coupling with thermospheric dynamics and/or auroral heating.
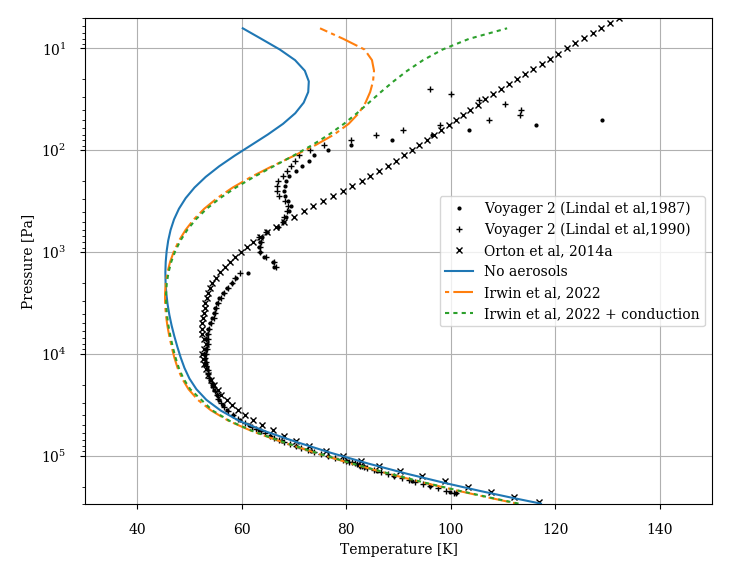
Figure 1: Temperature profiles from our simulations and from observations [3,4,16] at the same latitude and solar longitude of Voyager 2 measurements. Blue curve corresponds to a simulation without aerosols, orange curve aerosolsmodel from [17] is added, and for green curve, we add a conduction from thermosphere with aerosols. Methane molar fraction profile from [14] is used on our simulations.
Acknowledgements
G. Milcareck, S. Guerlet and A. Spiga acknowledge funding from Agence Nationale de la Recherche (ANR) project SOUND, ANR-20-CE49-00009-01.
References
[1] Marley, M. S. and McKay, C. P. (1999). Icarus, 138.
[2] Fletcher, L. N., de Pater, I., Orton, G. S., Hofstadter, M. D., Irwin, P. G. J., Roman, M. T., and Toledo, D. (2020). Space Science Reviews, 216.
[3] Lindal, G. F., Lyons, J. R., Sweetnam, D. N., Eshleman, V. R., Hinson, D. P., and Tyler, G. L. (1987). Journal of Geophysics Research, 92.
[4] Lindal, G. F. (1992). Astronomical Journal, 103.
[5] Herbert, F., Sandel, B. R., Yelle, R. V., Holberg, J. B., Broadfoot, A. L., Shemansky, D. E., Atreya, S. K., and Romani, P. N. (1987). Journal of Geophysical Research, 92.
[6] Broadfoot, A. L., Atreya, S. K., Bertaux, J. L., Blamont, J. E., Dessler, A. J., Donahue, T. M., Forrester, W. T., Hall, D. T., Herbert, F., Holberg, J. B., Hunten, D. M., Krasnopolsky, V. A., Linick, S., Lunine, J. I., Mcconnell, J. C., Moos, H. W., Sandel, B. R., Schneider, N. M., Shemansky, D. E., Smith, G. R., Strobel, D. F., and Yelle, R. V. (1989). Science, 246.
[7] Orton, G. S., Fletcher, L. N., Moses, J. I., Mainzer, A. K., Hines, D., Hammel, H. B., Martin- Torres, F. J., Burgdorf, M., Merlet, C., and Line, M. R. (2014). Icarus, 243.
[8] Fletcher, L. N., de Pater, I., Orton, G. S., Hammel, H. B., Sitko, M. L., and Irwin, P. G. J. (2014). Icarus, 231.
[9] Greathouse, T. K., Richter, M., Lacy, J., Moses, J., Orton, G., Encrenaz, T., Hammel, H. B., and Jaffe, D. (2011). Icarus, 214.
[10] Li, C., Le, T., Zhang, X., and Yung, Y. L. (2018). Journal of Quantitative Spectroscopy and Radiative Transfer, 217.
[11] Guerlet, S., Spiga, A., Sylvestre, M., Indurain, M., Fouchet, T., Leconte, J., Millour, E., Wordsworth, R., Capderou, M., Bézard, B., and Forget, F. (2014). Icarus, 238.
[12] Guerlet, S., Spiga, A., Delattre, H., and Fouchet, T. (2020). Icarus, 351.
[13] Madeleine, J. B., Forget, F., Millour, E., Navarro, T., and Spiga, A. (2012). Geophysical Research Letters, 39.
[14] Lellouch, E., Moreno, R., Orton, G. S., Feuchtgruber, H., Cavalié, T., Moses, J. I., Hartogh, P., Jarchow, C., and Sagawa, H. (2015). Astronomy and Astrophysics, 579.
[15] Rizk, B. and Hunten, D. M. (1990). Icarus, 88.
[16] Lindal, G. F., Lyons, J. R., Sweetnam, D. N., Eshleman, V. R., Hinson, D. P., and Tyler, G. L. (1990). Geophysical Research Letters, 17.
[17] Irwin, P. G. J., Teanby, N. A., Fletcher, L. N., Toledo, D., Orton, G. S., Wong, M. H., Roman, M. T., Perez-Hoyos, S., James, A., and Dobinson, J. (2022). arXiv e-prints.